High-Q factor photonic crystal cavities with cut air holes [Invited]
Download: 809次
Planar photonic crystal (PPC) cavities fabricated in dielectric slabs have a variety of advantages to resonantly control light-matter interactions and light wave propagation[1
In the past decades, a number of designs have been reported to realize PPC nanocavities with a high factor and small [19
Here, we propose that cutting two air holes in the PPC into semicircles is another important concept for realizing and optimizing cavities, which shows advantages over the cavities designed with missed or moved air holes in a higher factor and smaller . As examples, we present the formations of decent cavities by simply cutting two air holes either in a PPC lattice or in a PPC waveguide, as well as the great optimization of the typical -type PPC cavity by cutting two air holes at the cavity edges without movement of air holes.
Cavity formed by cutting air holes in PPC lattice - We first discuss the formation of a cavity by cutting a couple of air holes in a PPC lattice with periodic air holes, without missing or moving air holes. The PPC lattice is schematically displayed in Fig.
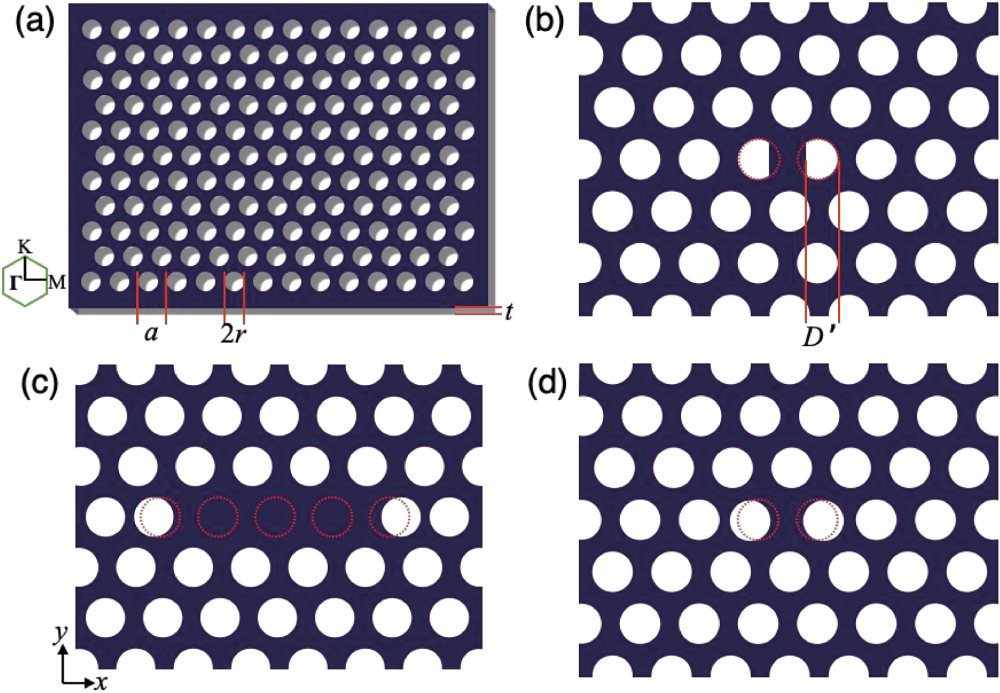
Fig. 1. (a) Schematic of the employed PPC lattice with a triangular lattice of air holes in a silicon slab. Inset: the reciprocal lattice of the PPC lattice. (b)–(d) Zoomed structures around the defect regions of the (b) , (c) , and (d) -type PPC cavities. The initial positions and profiles of the air holes in the PPC lattice are denoted by dashed red circles.
To assess the resonant performances of the -type PPC cavity, we also consider the resonant modes in widely studied - and -type PPC cavities with the same air hole lattice, whose structures are shown in Figs.
Resonant modes of -type PPC cavities with different are then studied. The highest factor of the fundamental resonant mode is obtained from the cavity with . This resonant mode locates at the wavelength of 1461.9 nm and has a factor of 200,500 and a . Compared with the optimized - and -type cavities mentioned above, the optimized -type cavity has the highest factor and the smallest . Figure

Fig. 2. (a) Electric field distributions ( and ) of the fundamental resonant mode in a -type cavity with . (b) The profiles of the resonant modes in , , and -type cavities over the cavity centerline along the direction (solid lines) and fitted curves calculated from the product of a Gaussian envelope function and a sinusoidal wave (dashed lines). (c) The factor, , , and resonant wavelength of the fundamental resonant modes calculated from the -type cavities with a varied .
The main factor degrading factors in PPC cavities is the vertical radiation loss because the total internal reflection of the slab could not be satisfied for the light components with small in-plane wave vectors (in the range between 0 and , where is the wavelength of light in air). In the - and -type cavities, to improve the factors, moving air holes near the cavity edge is required, which extends the cavity’s physical length to make the mode have more components with large in-plane wave vectors. However, as shown in Figs.
To illustrate this, we fit the fundamental resonant modes of the optimized , , and -type cavities with curves corresponding to the products of a sinusoidal wave and a Gaussian function[24], as shown in Fig.
Note, for these , , and -type PPC cavities, modifying air holes in the outer layers around the defects could improve the factor further by another one order of magnitude[25
We also study the dependences of the factor, , , and resonant wavelength of the fundamental resonant mode on in the -type cavities, as shown in Fig.
For the cavities with , the factor of the fundamental resonant mode decreases gradually as is reduced from and reaches the smallest value for . Compared with the cavities with , the physical lengths of the cavity defects in these cavities with a narrower are much larger, and the in-plane wave vectors of the modes are large enough to ensure them being away from the light cone. However, the narrower width of causes the mode distribution to have smaller values outside the cavity edge () than that required for a standard Gaussian envelope, which causes strong mode scattering from the air holes outside the cavity edge. Note, in the case of , the -type cavity evolves into a cavity with two missed air holes, i.e., the -type cavity. The factor is as low as 1500 because the mode profile strongly deviates from the ideal Gaussian envelope, which is the same mechanism for the low factor in the standard -type cavity without moved air holes[31]. In addition, compared with the optimized -type cavity, the cavity with a smaller has a larger physical dimension, which results in a larger . In the cavity with , the is about . For the -type cavities with a different , the factor of has the similar trend to that of the factor due to the small variation of the . It indicates that the optimized cavity for the maximum factor also supports the highest factor for enhancing light-matter interactions. However, the resonant wavelengths of the fundamental modes vary strongly, as shown Fig.
In the previously reported PPC cavities, such as - and -type cavities, besides the fundamental resonant mode, it is also possible to form higher-order resonant modes with higher optical frequencies, though the factors are normally smaller than that of the fundamental resonant mode due to the smaller in-plane wave vectors[30]. In realistic applications of PPC cavities, this multi-mode behavior is not preferred. For instance, in micro-lasers based on PPC cavities, the multiple resonant modes would compete for the gain and subsequently increase the laser threshold, which also hinders the single-mode operation of the laser. To employ PPC cavities in optical sensors, the multiple modes limit the sensing range when the shift of resonant wavelength is utilized to monitor the parametric variations. As discussed above, because the physical length of the defect in the -type cavity is very small, especially for the case with a large , the in-plane wave vector of the fundamental resonant mode is very small. For the higher-order electromagnetic mode supported by the cavity defect, which has even smaller in-plane wave vectors and higher optical frequencies, it is difficult for it to form a resonant mode with a moderately high factor. Because the modes are even closer to the light cone in the energy-momentum space, hence, it is expected that the ultracompact -type cavity has the single-mode operation. From our calculations, for the cavity with , which is optimized for the fundamental resonant mode, the higher-order resonant mode cannot be convergent in the mode simulation. This single-mode property enables the -type cavity to have potentials in the single-mode lasing, large range optical sensing, and deterministic single photon sources. Of course, by narrowing the to allow a longer cavity length, a higher-order resonant mode would be present. For example, in a cavity with , a higher-order resonant mode with a factor of 1800 and a of is obtained at the wavelength of 1452.3 nm. Note that it is more than 140 nm away from the fundamental resonant mode (at the wavelength of 1594.2 nm), which could guarantee the single-mode operation in a large wavelength range.
Inducing a cavity in PPC waveguide with cut air holes - A PPC waveguide, with a line defect of missed air holes, is another important base structure for realizing PPC cavities that represent advantages over the cavities directly designed from the PPC lattice. To generate a cavity from the PPC waveguide, modifications of a section of the waveguide are normally exploited to induce a varied effective refractive index, which forms mode gap regions in the photonic band. Consequently, cavity modes are created below the transmission band of the PPC waveguide. In the cavities derived from the PPC waveguide, along the direction of the line defect in the waveguide, the resonant mode confinements are much gentler due to the absence of air holes, which is different from the cavity based on a PPC lattice. This makes the electric-field profile of the cavity mode close to the ideal Gaussian curve, promising high factors[24]. In addition, inducing a cavity based on a PPC waveguide could facilitate the coupling of the resonant modes directly. For the cavity originated from a PPC lattice, an extra side coupled PPC waveguide is normally required to access the resonant modes[32].
The previously reported modifications of PPC waveguides for generating cavities include setting different horizontal lattice constants over a few periods to form a heterostructure[23] and outward moving air holes adjacent to the line defect of the waveguide to locally tune the waveguide width[21]. Here, we illustrate that simply cutting two air holes on opposite sides of the PPC waveguide’s line defect could form a PPC cavity with high resonant modes. The structure is schematically shown in Fig.
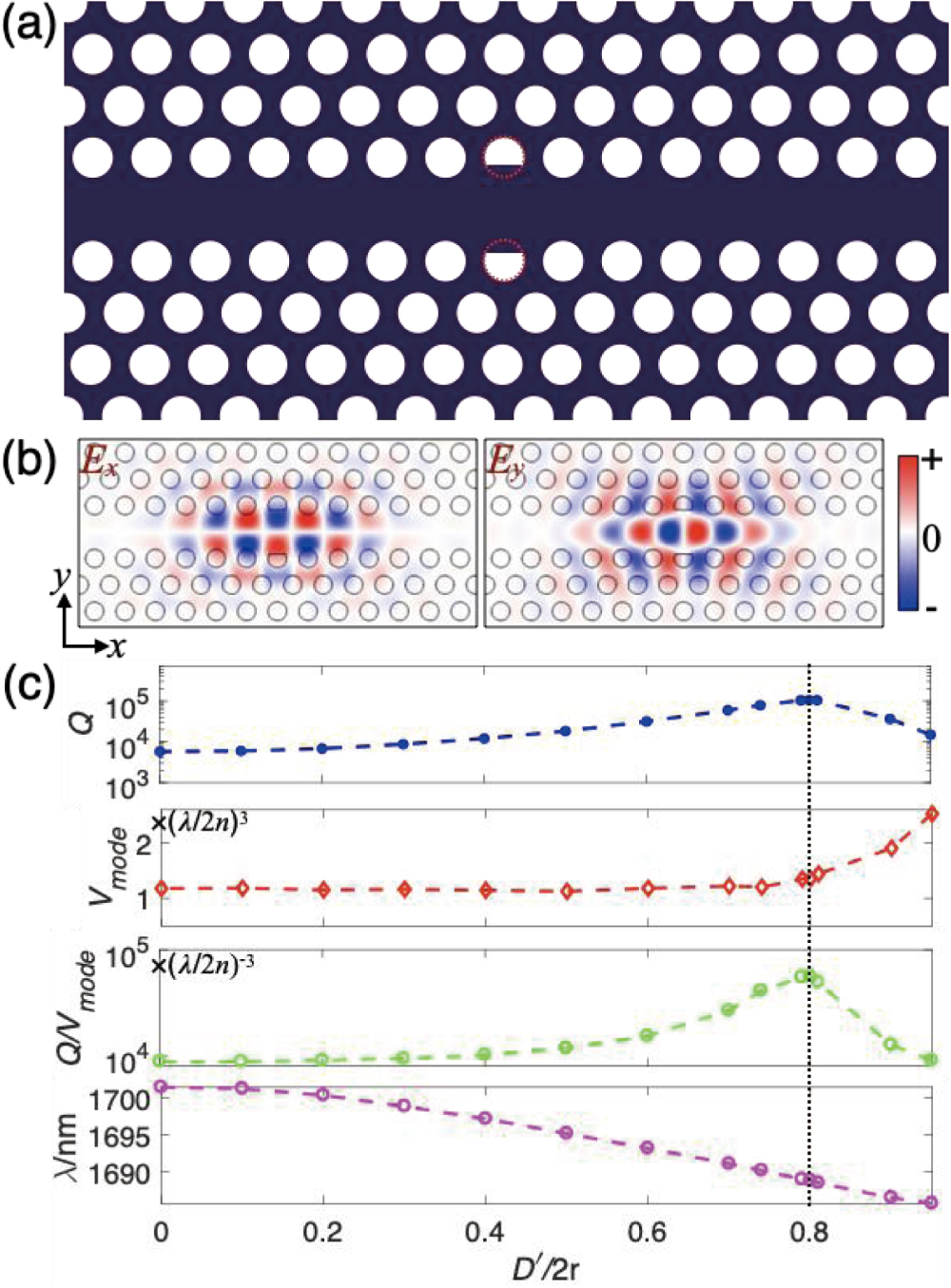
Fig. 3. (a) Schematic structure of the cavity induced by cutting two air holes adjacent to the line defect of a PPC waveguide. The dashed red circles represent the initial profiles of the cut air holes. (b) The electric field distributions ( and ) of the fundamental resonant mode with . (c) The factor, , , and resonant wavelength of the fundamental resonant modes versus .
Instead of cutting the two air holes, the cavity induced by outward moving the two air holes away from the line defect of the PPC waveguide is studied as well for comparison. The resonant mode with the highest factor is obtained from a cavity with a displacement of , and the factor is 96,700, which is smaller than that obtained from the cavity with cut air holes. This could also be explained by the flat interface of the semicircles facing the mode distributions, which more relaxes the scattering loss induced by the impedance mismatch. In addition, we also consider the PPC cavity formed by cutting more air holes adjacent to the PPC waveguide defect. Even higher factors are obtained than that with two cut air holes due to the wider mode distribution, while the becomes larger.
Figure
Optimizing L3 PPC cavity with cut air holes - We further demonstrate that the strategy of cutting air holes could also be employed to optimize the factor of the widely studied -type PPC cavity, where is the number of missed air holes in the defect region. In an -type PPC cavity, to improve factors, outward moving the nearest-neighbor air holes at the cavity edge is essential to make the electric field profile vary more gently, as shown in Fig.

Fig. 4. (a) Schematic structure of the -type cavity with cut air holes at the cavity edge. The dashed red circles represent initial positions and profiles of the air holes in the PPC lattice. (b) The electric field distributions ( and ) of the fundamental resonant mode with . (c) The factor, , , and resonant wavelength of the fundamental resonant mode versus .
Figure
By further cutting the two air holes with , the factor decreases gradually. This could be attributed to the deviation of the mode profile over the cavity centerline from the ideal Gaussian envelope function, similar to that discussed for the -type cavity. An abrupt change of the mode profile still remains at the cavity edge. For the case with , the factor decreases to a relatively small value of 24,900. Note that it is still higher than that in the standard -type cavity. It could be understood that the -type cavity with evolves into a standard -type cavity, which has larger in-plane wave vector benefitting from the longer physical defect length[30]. Hence, the vertical scattering for the resonant mode in the -type cavity should be smaller than that in the -type cavity, promising a higher factor.
In conclusion, we have demonstrated that simply cutting air holes in PPC to semicircles could be considered as a new strategy to form and optimize PPC cavities. Three examples are described. In the formations of PPC cavities, by simply cutting two adjacent air holes in a PPC lattice or two air holes on the opposite sides of a PPC waveguide, resonant modes with a factor exceeding are obtained. In the optimization of the PPC cavity, by cutting the two air holes at the edge of an -type cavity, the factor is optimized by more than 20 times, while is almost constant. Results of these three examples also indicate that the cavities with cut air holes have superiorities over cavities with missed or moved air holes in the higher factor and smaller . In the cavities with missed or moved air holes, the air holes around the cavity edges are circularly shaped. By contrast, the cut air holes have flat sides facing the strong mode distribution, which could relax the impedance mismatch at the interfaces of air holes and the silicon slab more. Hence, cut air holes could enable much gentler mode confinement in PPC cavities. The cut air hole as a structure parameter provides a new strategy to design other PPC devices, such as PPC waveguides with engineered dispersions and low transmission losses.
[1]
[2]
[3]
[4]
[5]
[6]
[7]
[8]
[9]
[10]
[11]
[12]
[13]
[14]
[15]
[17]
[18]
[19]
[20]
[22]
[23]
[24]
[25]
[26]
[28]
[29]
[30]
[31]
[32]
Liang Fang, Xuetao Gan, Jianlin Zhao. High-Q factor photonic crystal cavities with cut air holes [Invited][J]. Chinese Optics Letters, 2020, 18(6): 063603.