Directly generating vortex beams in the second harmonic by a spirally structured fundamental wave
Download: 1046次
1. Introduction
It is well known in optics that circularly polarized photons carry angular momentum of , and this is called spin angular momentum, which was firstly, to the best of our knowledge, reported by Beth in 1935[1]. Also, linearly polarized waves with a helical phase distribution can carry orbital angular momentum (OAM) of per photon, where and denote the topological charge (TC) and azimuth angle, respectively. The vortex beams carrying OAM exhibiting a donut‐like‐shaped intensity pattern with no limits on TC have been extensively applied in optical communication[2–
In this paper, we demonstrate experimentally a simple and efficient method to generate an OAM beam in SH by utilizing an FW beam with spirally modulated phase incident into a homogenous medium. In the experiments, the phase profile of the FW carrying positive and negative integers or fractional OAM is investigated. This study reveals that the radii of ring‐shaped SH vortex beams become larger with the increase of the absolute value of the TC of the structured FW. Nevertheless, the circular intensity pattern of the SH vortex beam transforms to elliptical and tilts into the opposite direction when the positive fractional TC is turned to negative. Moreover, the elliptical patterns of SH vortex beams are stretched along a certain direction by adding the absolute value of the TC of the structured FW.
2. Methods
Supposing that the light beam propagates along the axis, a Gaussian beam is reflected by an SLM, and thus the phase information is imprinted into the input beam, so the structured FW can be rewritten as the following:
The SH radiation can be highly efficiently generated under the condition of . The rightmost exponential term stands for the OAM of SH radiation. In our experiment, the structured FW propagates along the birefringent phase‐matching direction for satisfying the momentum conservation between the wave vectors of the SH and the FW. Therefore, Eq. (2) can be simplified to the following:
3. Results and Discussion
In the experiment depicted in Fig. 1, a Gaussian beam is delivered from a mode-locked (Nd:YAG) nanosecond laser with the wavelength of 1064 nm.The duration of the output pulse is approximately 3 ns, and the repetition rate is 20 Hz. A half‐wave plate (HWP) and a Glan–Taylor (G–T) prism are used to control the intensity and polarization of the Gaussian beam. After being expanded and collimated, the Gaussian beam is modulated and structured by an SLM. The SLM used in our experiment has a resolution of pixels. The Gaussian beam whose beam waist is approximately 500 µm is imaged by a system to imprint the structured FW to a homogenous 5% (mole fraction) crystal ( in dimensions) along the birefringent phase‐matching direction. According to the Sellmeier equation, the angle between the propagation direction ( axis) and optic axis of the crystal is 75°. A shortpass filter is placed behind the nonlinear medium to obstruct the FW. Finally, the SH beam pattern carrying OAM is projected on a screen that is far away from the medium 12 cm and is captured by a camera.
To manifest the relationship between the generated SH vortex beams and the structured FW beams, we firstly imprint the structured FW beams with fan‐like phase profiles onto the nonlinear crystal. The phase profiles of the structured FWs are plotted in Figs. 2(a1)–2(a6), in which the phase value is modulated in the range of 0 to , and the modulated period in Eq. (1) is chosen as 91.4 µm. As shown in Figs. 2(a1)–2(a3), the cycle index that phase swept from 0 to is the same as the number of integral TC of the phase masks. Nevertheless, as shown in Figs. 2(a4)–2(a6), phase‐drifting occurs when the TC is a non‐integer. The second row in Fig. 2 represents the simulated SH patterns based on Eq. (3), which are close to the experimentally generated SH vortex patterns, as shown in the third row in Fig. 2. When the TC of the structured FW beam is varied from one integer () to another one (), it is obvious that the intensity profiles shown in Figs. 2(b1)–2(b3) have a rotationally symmetric multiple‐ring structure with a dark core at the center. The diameter of dark cores increases with the increase of the TC, and the corresponding SH intensity patterns are shown in Figs. 2(c1)–2(c3). Furthermore, despite the TC of the FW beam shown in Fig. 2(a4) being fractional (), a rotationally symmetric multiple‐ring pattern with a dark core at the center is still observed from Fig. 2(c4). Because the TC of the SH vortex beam is an integer, the value of OAM is exactly twice that of the input FW beam (). As the TC of FW beam further increases to , the first high‐intensity ring shown in Fig. 2(b5) breakes into three brighter spots in a relatively darker background, and the second high-intensity ring is also visibly distorted. For , the brighter spot originally formed in the second high‐intensity ring has moved inward to join the former three brighter spots in the first intensity ring. The recorded patterns shown in Figs. 2(c5) and 2(c6) verify the variation of intensity distribution and agree with the simulation. It is confirmed that the intensity profiles of the SH vortices with integer TC keep a rotationally symmetric multiple‐ring structure, whereas fractional TC can break and disorganize such symmetric structures to asymmetric ones.
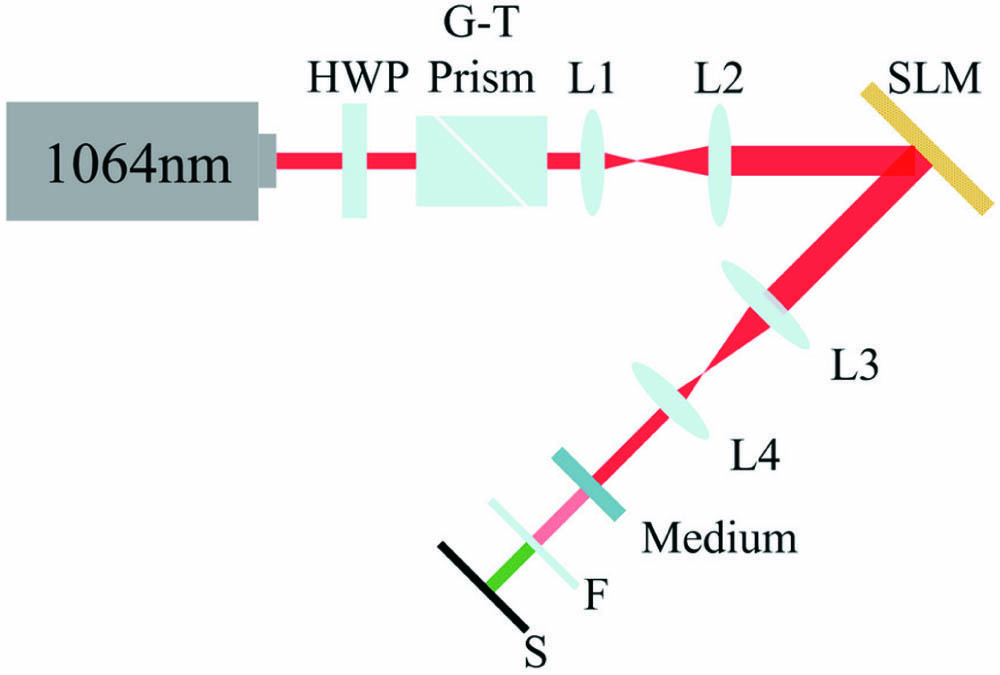
Fig. 1. Schematic of the experimental setup. HWP, half-wave plate; G-T prism, Glan–Taylor prism; L1–L4, lens with the focal length of 50, 100, 200, and 50 mm, respectively; medium, 5% (mole fraction) magnesium-oxide-doped periodically poled lithium niobate (MgO:PPLN); F, filter; S, screen.

Fig. 2. Generation of the SH vortex patterns carrying different l2, where l2 = 2l1, and l1 is the TC of the structured FW. The first row is the phase masks of the FW beams with different TCs for (a1) l1 = 1, (a2) l1 = 2, (a3) l1 = 3, (a4) l1 = 2.5, (a5) l1 = 2.7, and (a6) l1 = 2.9, respectively. The second and third rows show the corresponding simulation and experimental intensity profiles of the SH vortex with different TCs for (c1) l2 = 2, (c2) l2 = 4, (c3) l2 = 6, (c4) l2 = 5, (c5) l2 = 5.4, and (c6) l2 = 5.8, respectively. In this case, the obliquity factor is constant (a = 1).
We further consider the generation of ellipse vortex beams, in which the azimuthal angle changes according to . The obliquity factor has a strong influence on the intensity profile of the SH vortex beams. The intensity profiles of the SH vortices are transformed from circular into elliptical once obliquity factor is not equal to one. The phase profiles of the FWs with respectively, are arrayed in the first row of Fig. 3, and the phase varies continuously from 0 to . The twisted distortion is easily observed in spiral phase structures by changing of the obliquity factor . Accompanying the increase of the obliquity factor, the covered area of twisted distortion grows up in size whether the numeric type of the obliquity factor is an integer or fraction. The simulation and experimentally generated patterns of the ellipse vortex beams are demonstrated in the second and the third rows of Fig. 3, respectively. The simulations and the experiments are in good agreement with each other. It can be seen that the rotationally symmetric multiple‐ring shape of the SH vortex shown in Fig. 3(b1) is broken and transformed into ellipse when the obliquity factor is not equal to one, as shown in Figs. 3(b2)–3(b6). The inner bright symmetric ring of the SH vortex beam shown in Fig. 3(b1) splits into two separate brighter spots, and the circular shape of the dark core transforms into elliptical, as shown in Fig. 3(b2). Furthermore, the intensity profiles with high-order obliquity factors shown in Figs. 3(b3)–3(b6) are exhibited as two separate brighter spots. Meanwhile, the central dark core originating from Fig. 3(b1) evolves and degenerates into multiple discrete dark cores, with the location of dark cores keeping symmetry with respect to the central point of the light field. Interestingly, when the obliquity factors are reciprocal with each other, e.g., and , corresponding to Figs. 3(b2) and 3(b4), the orientation of the alignment of dark cores in the two cases is perpendicular to each other. That is because the spiral phase gradient has a relative shift of 90 deg for both cases, which had been analyzed in Ref. [28].

Fig. 3. Intensity patterns of the SH vortices with different obliquity factors. The first row denotes the phase profiles of the FW beams with different obliquity factors for (a1) a = 1, (a2) a = 2, (a3) a = 4, (a4) a = 0.5, (a5) a = 1.5, and (a6) a = 4.5, respectively. The TC l1 = 2 is fixed in this case. The simulation and experimental intensity patterns of the SH wave are arranged and displayed in the second and the third rows, respectively.
To further reveal the conversion rule of OAM in the nonlinear frequency conversion process, we also explore the behaviors of the OAM conservation in the SH generation process by switching the sign of the optical OAM or the obliquity factor, as shown in Fig. 4. When changing the sign of TC of the FW beam with from to , the rotation of the elliptical SH patterns in Figs. 4(a) and 4(b) turns counterclockwise. The tilted orientation maintains its symmetry with respect to the vertical axis. The same performance is also observed in the cases shown in Figs. 4(c) and 4(d), in which the sign of the obliquity factor is switched from to with the fixed input OAM . Due to the negative obliquity factor, as shown in Fig. 4(d), the spiral phase gradient becomes negative and results in the opposite orientation of alignment of dark cores in contrast with the case of in Fig. 4(c). Besides, the SH generation processes possess the OAM conservation regardless of the sign of input OAM or obliquity factor. It is interesting that the number of the TC of SH vortices is conveniently and immediately read when the obliquity factor is unequal to one. For clarity, arrows shown in Fig. 4 mark the number and orientation of the dark cores. We can deduce the positive or negative value of TC of SH vortices upon the number and orientation of arrows. The OAM of the SH beam is twice as large as the input OAM of the structured FW.
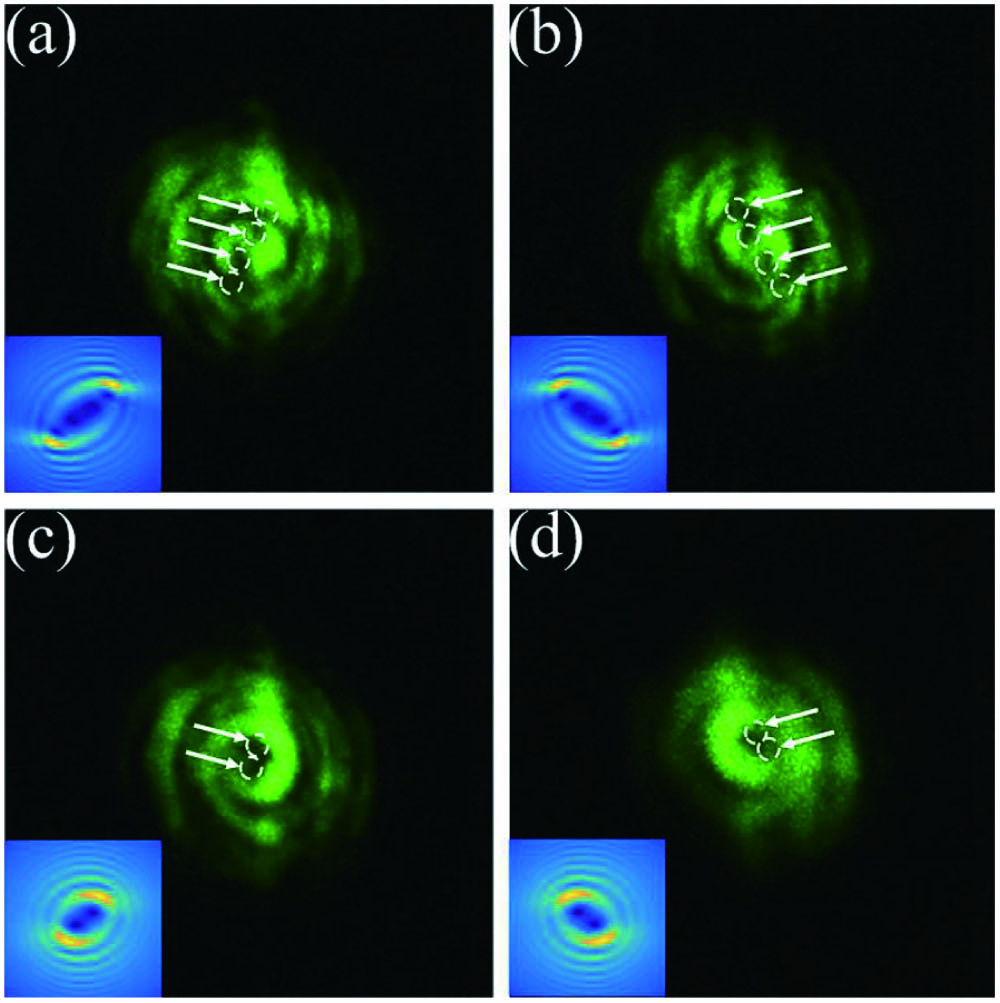
Fig. 4. Experimental comparison on the intensity patterns of generated SH waves by switching the sign of the TC of the structured FW beam and the obliquity factor, respectively. (a)–(d) Corresponding to cases of input OAM and obliquity factors that are l1 = +2, a = 3, l1 = −2, a = 3, l1 = 1, a = +2, and l1 = 1, a = −2, respectively. The insets are the corresponding simulation of SH vortex beams. The experiments are in good agreement with the simulations.
In addition, the conversion efficiency of frequency‐doubling process based on pump power carrying different OAM states and the SH signal power is analyzed and shown in Fig. 5. Obviously, the maximum conversion efficiency is 1.14% for SH generation, which is achieved without OAM imprinting. With the increase of the input OAM states, the conversion efficiency of the SH generation goes down dramatically. As shown in Fig. 5, the conversion efficiencies of 0.44%, 0.37%, and 0.35% are obtained experimentally at the OAM states , respectively, which is consistent with the theoretical analysis based on the relationship between the conversion efficiency and the fundamental power density of input FW beam , , where and denote the average power and the cross section of the structured FW beam, respectively. The decreased conversion efficiency is attributed to the increased cross section of the FW after the introduction of OAM. In our experiment, an input OAM state carrying a higher integer TC has a multiple‐ring‐shaped intensity profile with a bigger diameter, which results in a lower fundamental power density.

Fig. 5. Conversion efficiency of SH waves pumped by different OAM states with the fixed obliquity factor a = 1.
4. Conclusion
We have experimentally demonstrated the generation of vortex beams in SH by modulating the phase of the FW directly incident into a homogenous nonlinear crystal. The phase structure of the FW is dynamically controlled by an SLM, which allows us to flexibly and independently control the OAM state of the generated SH beams. In our experiments, both circular and elliptical vortex beams were investigated, and the universal conservation rule of OAM in the SH generation process was verified directly as the obliquity factor does not equal one. Our approach can generate any demanded SH signals with arbitrary OAM, which may be useful in OAM‐related physical branches such as quantum optics, micromanipulation in particles, and nonlinear optics.
[1] R. A. Beth. Direct detection of the angular momentum of light. Phys. Rev., 1935, 48: 471.
[2] J. Wang, J. Y. Yang, I. M. Fazal, N. Ahmed, Y. Yan, H. Huang, Y. Ren, Y. Yue, S. Dolinar, M. Tur. Terabit free-space data transmission employing orbital angular momentum multiplexing. Nat. Photon., 2012, 6: 488.
[3] B. Nenad, Y. Yang, Y. Ren, T. Moshe, K. Poul, H. Hao, A. E. Willner, R. Siddharth. Terabit-scale orbital angular momentum mode division multiplexing in fibers. Science, 2013, 340: 1545.
[4] L. Chen, R. K. Singh, A. Dogariu, Z. Chen, J. Pu. Estimating topological charge of propagating vortex from single-shot non-imaged speckle. Chin. Opt. Lett., 2021, 19: 022603.
[5] Y. Liu, X. Yan, J. Wu, B. Zhu, Y. Chen, X. Chen. On-chip erbium-doped lithium niobate microcavity laser. Sci. Chin. Phys. Mech., 2021, 64: 234262.
[6] D. G. Grier. A revolution in optical manipulation. Nature, 2003, 424: 810.
[7] M. Padgett, R. Bowman. Tweezers with a twist. Nat. Photon., 2011, 5: 343.
[8] Z. Shen, L. Su, X. C. Yuan, Y. C. Shen. Trapping and rotating of a metallic particle trimer with optical vortex. Appl. Phys. Lett., 2016, 109: 241901.
[9] A. Nicolas, L. Veissier, L. Giner, E. Giacobino, D. Maxein, J. Laurat. A quantum memory for orbital angular momentum photonic qubits. Nat. Photon., 2014, 8: 234.
[10] F. Severin, J. Alexander, B. Stefan, R. M. Monika. Spiral phase contrast imaging in microscopy. Opt. Express, 2005, 13: 689.
[11] S. S. R. Oemrawsingh, J. A. W. van Houwelingen, E. R. Eliel, J. P. Woerdman, E. J. K. Verstegen, J. G. Kloosterboer, G. W. Hooft. Production and characterization of spiral phase plates for optical wavelengths. Appl. Opt., 2004, 43: 688.
[12] N. R. Heckenberg, R. McDuff, C. P. Smith, A. G. White. Generation of optical phase singularities by computer-generated holograms. Opt. Lett., 1992, 17: 221.
[13] Y. Pan, X. Gao, R. Ma, C. Tu, Y. Li, H. Wang. Tunable azimuthally non-uniform orbital angular momentum carried by vector optical fields. Chin. Opt. Lett., 2020, 18: 122601.
[14] B. Y. Wei, S. Liu, P. Chen, S. X. Qi, Y. Zhang, W. Hu, Y. Q. Lu, J. L. Zhao. Vortex Airy beams directly generated via liquid crystal q-Airy-plates. Appl. Phys. Lett., 2018, 112: 121101.
[15] X. Cai, J. Wang, M. J. Strain, B. Johnson-Morris, J. Zhu, M. Sorel, J. L. O’Brien, M. G. Thompson, S. Yu. Integrated compact optical vortex beam emitters. Science, 2012, 338: 363.
[16] B. Etienne, M. Naoki, M. Hiroaki, J. Saulius. Optical vortices from liquid crystal droplets. Phys. Rev. Lett., 2009, 103: 103903.
[17] H. Li, H. Liu, X. Chen. Nonlinear generation of Airy vortex beam. Opt. Express, 2018, 26: 21204.
[18] B. Alon, A. Ady. Generation of optical vortex beams by nonlinear wave mixing. Opt. Express, 2007, 15: 17619.
[19] C. Lin, Y. Chen, X. Li, L. Yang, R. Ni, G. Zhao, Y. Zhang, X. Hu, S. Zhu. Frequency-doubled vortex beam emitter based on nonlinear Cherenkov radiation. Chin. Opt. Lett., 2020, 18: 071902.
[20] Y. Wu, R. Ni, Z. Xu, Y. Wu, X. Fang, D. Wei, X. Hu, Y. Zhang, M. Xiao, S. Zhu. Tunable third harmonic generation of vortex beams in an optical superlattice. Opt. Express, 2017, 25: 30820.
[21] F. Kong, C. Zhang, F. Bouchard, Z. Li, G. G. Brown, H. K. Dong, T. J. Hammond, L. Arissian, R. W. Boyd, E. Karimi. Controlling the orbital angular momentum of high harmonic vortices. Nat. Commun., 2017, 8: 14970.
[22] G. Genevieve, L. Jonathan, K. T. Kim, T. J. Hammond, E. Frumker, R. W. Boyd, P. B. Corkum. Creating high-harmonic beams with controlled orbital angular momentum. Phys. Rev. Lett., 2014, 113: 153901.
[23] D. Gauthier, P. R. Ribič, G. Adhikary, A. Camper, C. Chappuis, R. Cucini, L. F. Dimauro, G. Dovillaire, F. Frassetto, R. Géneaux. Tunable orbital angular momentum in high-harmonic generation. Nat. Commun., 2017, 8: 14971.
[24] H. Jiang, X. Yan, H. Liang, R. Luo, X. Chen, Y. Chen, Q. Lin. High harmonic optomechanical oscillations in the lithium niobate photonic crystal nanocavity. Appl. Phys. Lett., 2020, 117: 081102.
[25] H. Li, H. Liu, X. Chen. Nonlinear vortex beam array generation by spatially modulated fundamental wave. Opt. Express, 2017, 25: 28668.
[26] H. Zhou, H. Liu, M. Sang, J. Li, X. Chen. Nonlinear Raman–Nath second harmonic generation of hybrid structured fundamental wave. Opt. Express, 2017, 25: 3774.
[27] H. Liu, J. Li, X. Zhao, Y. Zheng, X. Chen. Nonlinear Raman–Nath second harmonic generation with structured fundamental wave. Opt. Express, 2016, 24: 3774.
[28] G. H. Kim, H. J. Lee, J. U. Kim, H. Suk. Propagation dynamics of optical vortices with anisotropic phase profiles. J. Opt. Soc. Am. B, 2003, 20: 351.
Jun Li, Haigang Liu, Yan Li, Xianping Wang, Minghuang Sang, Xianfeng Chen. Directly generating vortex beams in the second harmonic by a spirally structured fundamental wave[J]. Chinese Optics Letters, 2021, 19(6): 060005.