Controllable photonic spin Hall effect with phase function construction
Download: 610次
1. INTRODUCTION
Photonic spin Hall effect (SHE), originating from the spin-orbit interaction and manifesting as spin-dependent splitting (SDS) [13" target="_self" style="display: inline;">–
To achieve more diverse applications, such as the optical computing of spatial differentiation realized via analyzing the orthogonal polarization states of light and the differential effect obtained through tuning the SDS [5], the controllable photonic SHE is highly demanded to flexibly manipulate the spin-polarized photons. Except for manipulating the SDS, extra phase modulation is also pursued for performing more operations on the spin components. To manipulate the SDS in the transverse and longitudinal directions simultaneously, the gradient phase combined with a conventional lens was used [21]. Moreover, the helical phase was integrated with the gradient phase to impose orbital angular momenta to the two spin components [22].
Flexible manipulation of the photonic SHE is always a challenge in practical applications. In the RVB-based tunable SDS, varying the thickness of the reflecting layered structure is the most commonly used method [7]. The graphene and graphene-like materials were employed to avoid changing the device structure, where the SDS could be dynamically tuned by varying the Fermi energy [23]. Unfortunately, limited by the naturally tiny SDS, the tuning range, which is usually on the order of a wavelength, is quite low. Hence, the SDS coming from the P-B phase with large manipulation ranges seems to be more attractive. By tailoring the space-variant P-B phase contributed by cylindrical vector beams (CVBs), the photonic SHE can also be tuned; however, it involves changing the structure of incident light beams, which is very difficult in practical applications [24]. Meanwhile, these conventional SHE manipulation methods make it hard to meet the requirement of system integration and extra phase modulation.
Metasurfaces, which enable engineering the spatial phases of light by defining the geometry, orientation, and arrangement of subwavelength-scale building blocks [2527" target="_self" style="display: inline;">–
In this work, we propose a controllable SHE based on phase function construction in which the phases (such as twisting phase and lens phases) with specific functional structures performing a coordinate translation are equivalent to integrating a gradient phase to the original phases, which can be used to realize controllable SDS in the momentum space. Meanwhile, with the original phases, the extra phase modulation of the two orthogonal spin components can be achieved simultaneously. To verify this mechanism, we fabricate a dielectric geometric P-B phase metasurface by writing spatially variant grooves in the silica glass to impose conjugate twisting phases to the two spin components. It is experimentally demonstrated that the SDS can be continuously manipulated in the visible region (three wavelengths of 633, 532, and 475 nm with the diffraction efficiencies of 98.72%, 95.05%, and 90.06% are chosen) by shifting the incident light beams. With the giant SDS, the polarization ellipticity is successfully measured. Moreover, the extra phase modulation is achieved under the action of twisting phase, with the result that the spin components of singular beams are separated and diffracted into specific intensity patterns. It is also demonstrated that P-B phase metasurface succeeds in detecting the topological charges and polarization orders of arbitrary singular beams [the vortex beams (VBs) with the topological charges of 1 and 2, the CVBs with the polarization orders of and 2, and the cylindrical vector vortex beams (CVVBs) with the topological charges and polarization orders of (1, 2) and (2, )]. These results confirm that the controllable SHE based on phase function construction can be used for manipulating the SDS and extra phase modulation, which may pave new avenues in spin-photonics applications.
2. THEORY AND PRINCIPLE
We consider a light beam paraxially propagating along axis , and the phase-correcting metasurface, located in the transverse (, ) plane, whose action is the spin-dependent modulation of the incident beam phase. If the incident beam with the transverse amplitude distribution crosses such a P-B phase metasurface, the beam’s complex amplitude transforms as , where and is the local optical axis direction of the metasurface, for the left (LCP) and for the right (RCP) circularly polarized beams, respectively. The spin-dependent phase gradient is one of the conventional methods to achieve SDS. For example, the SDS in the horizontal () direction can be realized if the function contains the summand with a certain constant parameter : The elementary deduction [Eqs. (
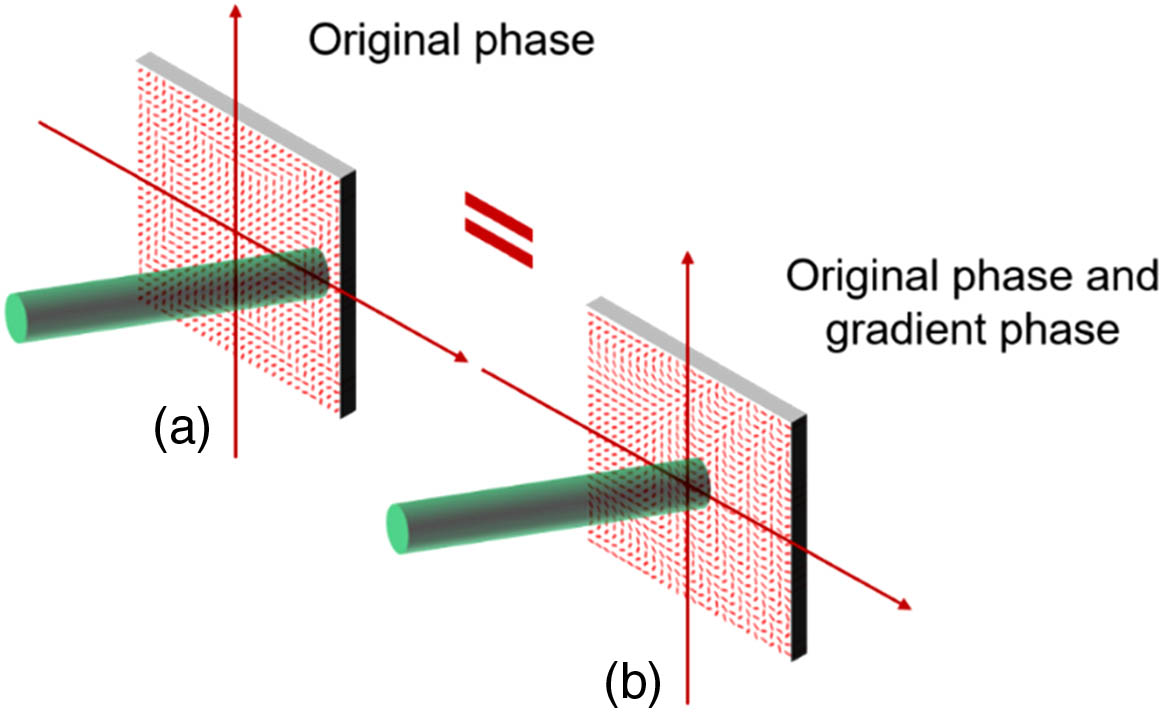
Fig. 1. Shifting the (a) incident position onto the P-B phase metasurface carrying the original phases is equivalent to (b) centrally incident on the P-B phase metasurface carrying the original phase and gradient phase.
With an LCP or RCP Gaussian beam incident, the output field can be expressed as where and are the simplified amplitude of LCP and RCP Gaussian beams. Hence, by properly designing the optical axis of metasurface under half-wave retardance, the above functions can be performed with P-B phase devices.
After that, we find that there are two typical phases that can meet the above functional structure. They are the twisting phase [39] and the dynamical phase lens [21], whose phase functions can be expressed as In the twisting phase , is the certain constant strength factor, and is the azimuthal angle. When , Eq. (

Fig. 2. (I) Illustration of the twisting phase adding together with the gradient phase. (a)–(c) Two-dimensional slow-axis orientation patterns of the twisting phase, gradient phase, and combination phase. (d)–(i) Phases introduced to the LCP and RCP light. (II) Illustration of the lens phase adding together with the gradient phase. (j)–(l) Two-dimensional slow-axis orientation patterns of the lens phase, gradient phase, and combination phase. (m)–(r) Phases introduced to the LCP and RCP light.
Hence, the P-B phase metasurfaces engineered with the twisting phase or lens phase can dynamically manipulate the SDS by shifting the incident beam with respect to the center of the metasurface.
Assuming that the linearly polarized Gaussian beam illuminates the metasurface carrying the twisting phase () with a lateral shift of , Eq. (
The extra phase modulation can be performed with the action of the twisting phase or the dynamic phase lens. According to previous work [39], the VB, which is modulated with a twisting phase that diffracts into a specific intensity pattern, can be used for topological charge recognition. Thus, we can manipulate the SDS by varying the position shift of incident light, and the topological charge recognition can be achieved with the modulation of twisting phases.
3. RESULTS AND ANALYSIS
3.1 A. Tunable SDS in the Geometric P-B Phase Metasurface
1. Relationship between SDS and Position Shift
To experimentally verify the proposed mechanism, we have fabricated a geometric P-B phase metasurface by writing spatially variant nanogrooves in the fused silica glass substrate, which can vary the optical axes distribution and impose conjugate twisting phases to the LCP and RCP light. The induced nanogrooves can modulate the refractive indices to form birefringence in the isotropic glass sample [40]. The directions of the local optical axes (fast and slow axes) are perpendicular and parallel to the grooves, respectively. The fabrication processing is given in detail in Appendix

Fig. 3. (a) Illustration of the Gaussian beam illuminating the dielectric P-B phase metasurface. (b) Captured picture of the fabricated metasurface. (c) Polariscopic analysis carried out by optical polarization imaging. (d)–(f) Measured SDS intensity (normalized) images of Gaussian beams at different wavelengths (633, 532, and 475 nm).
In the experiment, the metasurface is illuminated with a linearly polarized Gaussian beam, which is generated by a He-Ne laser (Thorlabs HNL210L-FC; the working wavelength, 633 nm; the maximum output power, 21 mW). The output beam is transversely split into LCP and RCP components by the metasurface, which is illustrated in the measured Stokes parameters at the transmission distance of 500 mm, as shown in Fig.
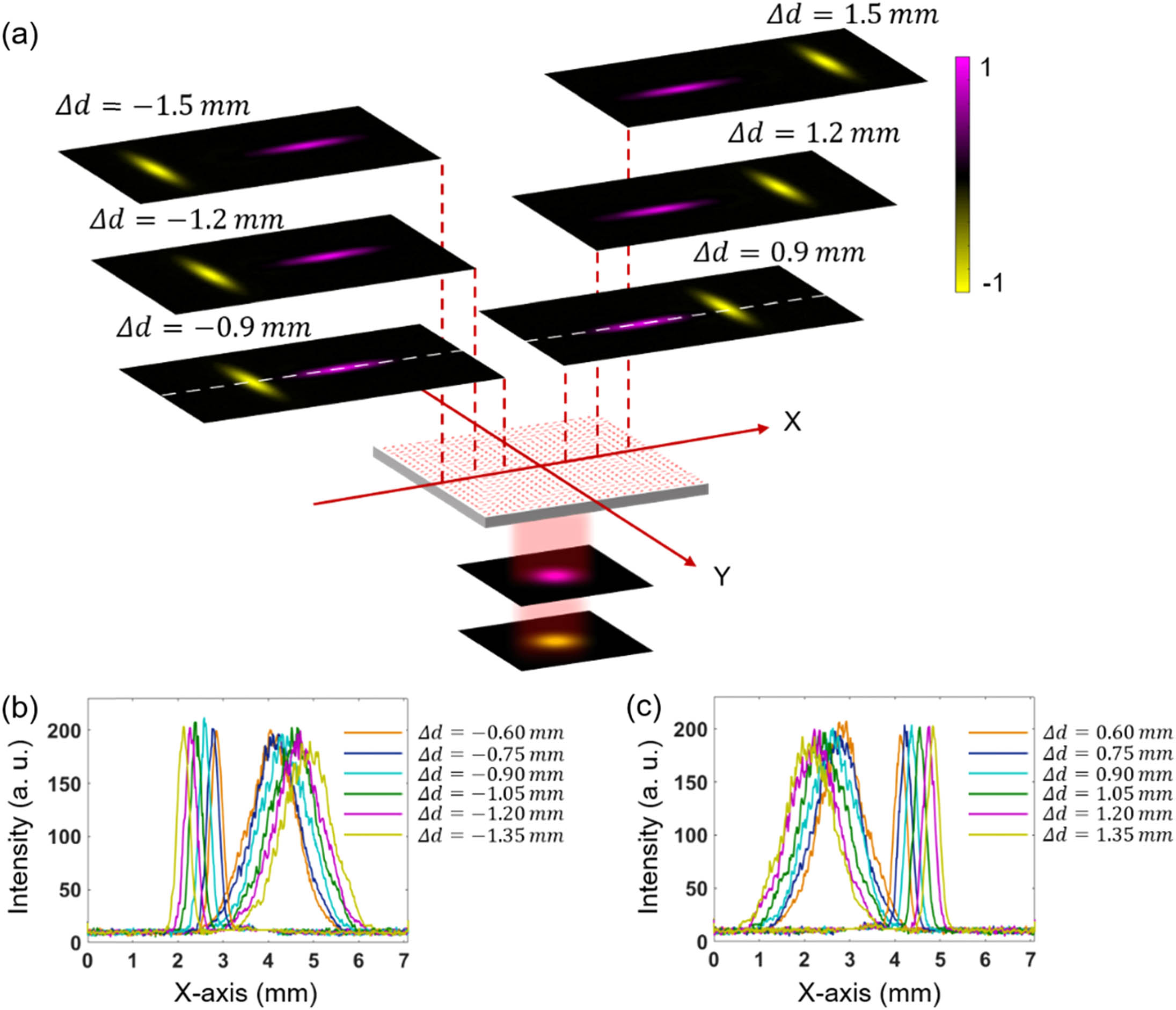
Fig. 4. (a) Measured Stokes parameters of the linearly polarized Gaussian beams at the incident position shifts of , and . (b) and (c) Intensity curves corresponding to the white dashed lines across the intensity images at the incident position shifts of , and .

Fig. 5. Measured intervals between LCP and RCP components with different incident position shifts at the transmission distance of 500 mm. Cal., calculated; Exp., experimental.
Owing to the P-B phase, the metasurface shows a dispersion-free characteristic in the visible region, which leads to a broadband working wavelength range. Figures
2. Polarization Ellipticity Detection Based on SDS
Once the spin components of the incident beam are separated, the intensity ratio () of the two components can be measured, which enables measuring the ellipticity [] and the handedness of polarized beams [20], where represents LCP (RCP), and represents linear polarization. Thus, the metasurface enables the polarization ellipticity detection of light by using the SDS. We test the polarization of Gaussian beams at the wavelength of 633 nm. The polarization state of the Gaussian beam is modulated by varying the angle () between the transmission axis of the polarizer and the fast axis of the quarter-wave plate (QWP). As shown in Fig.
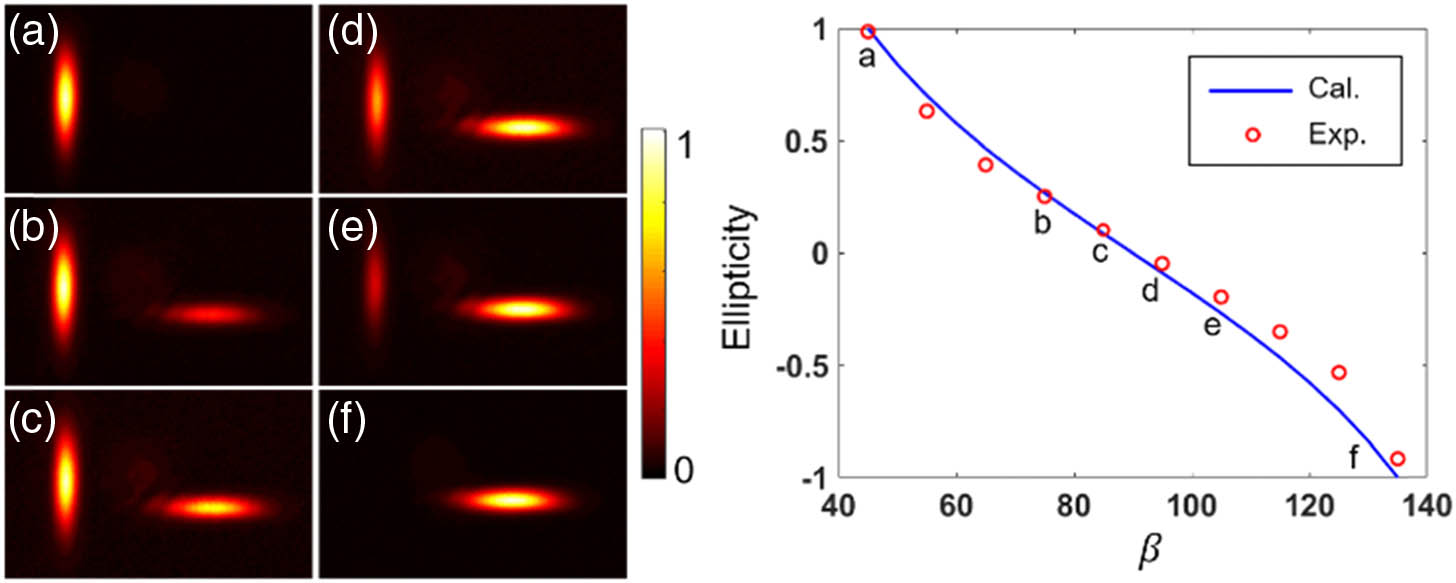
Fig. 6. Measured ellipticities versus the incident polarization (a function of ). (a)–(f) Optical intensity (normalized) profiles correspond to situations of , and 135° at the wavelength of 633 nm. Cal., calculated; Exp., experimental.
3.2 B. Extra Phase Modulation of the Twisting Phase and Singular Beam Detection
Moreover, the extra phase modulation of the twisting phase is performed and successfully used for detecting singular beams. To verify this, a VB illuminates the metasurface with an incident position shift of . Referring to the previous work [42], a polarizer, QWP, and conventional metasurface -plate () are combined to generate circularly polarized VB with the topological charge of 2, which is transformed into linearly polarized VB with another QWP, as shown in part (i) of Fig.
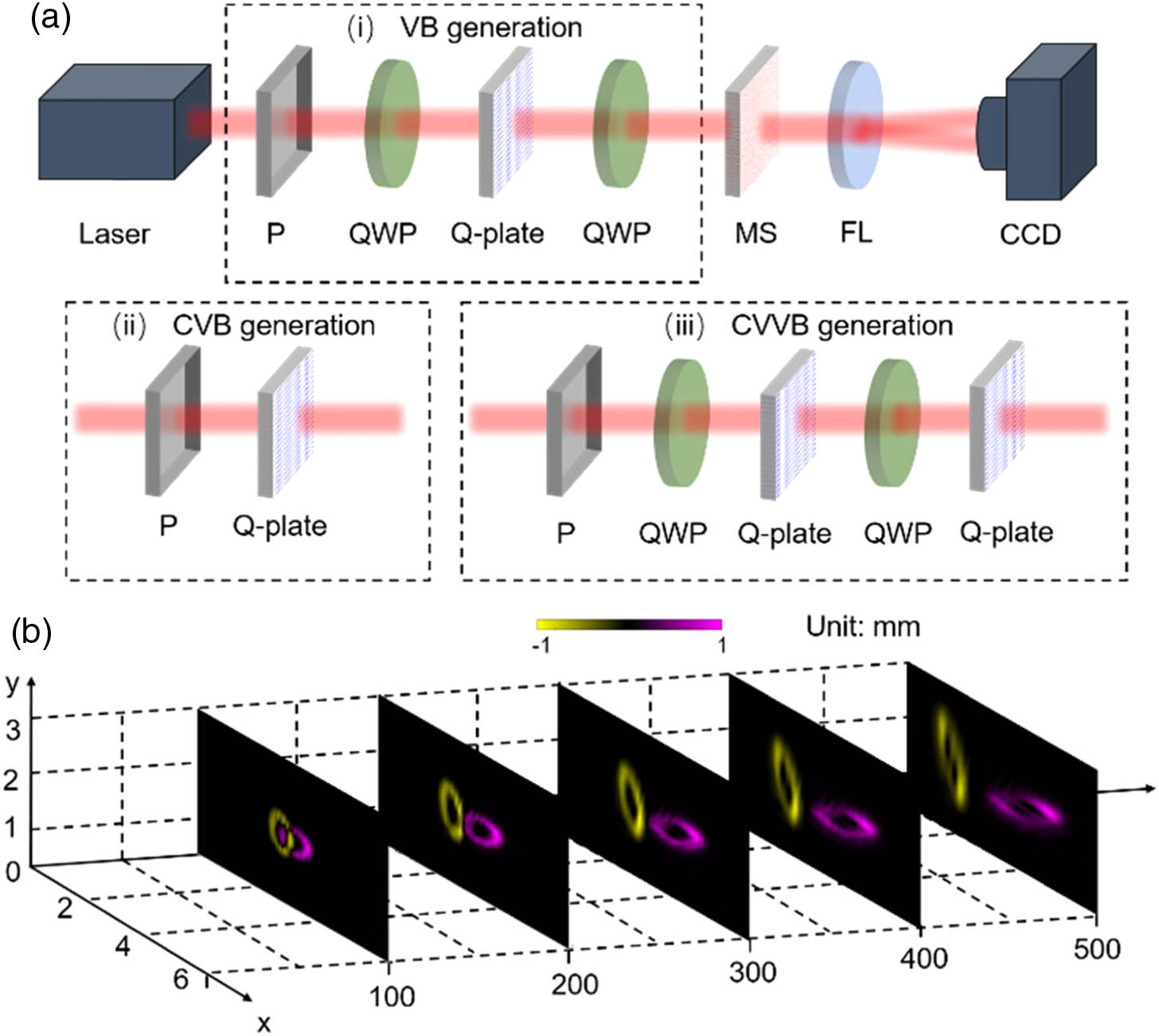
Fig. 7. (a) Schematic diagram of the experimental setups of arbitrarily singular beams detection based on the geometric P-B phase metasurface. P, polarizer; QWP, quarter-wave plate; MS, metasurface; FL, Fourier lens; CCD, charge-coupled device. (b) Measured parameters of the output beams with different transmission distances ( , 200, 300, 400, and 500 mm).
Because the beams with polarization singularities (CVB and CVVB) can be decomposed into two sub-VBs with orthogonal circular polarization states, the metasurface also enables detecting the polarization orders and topological charges of CVBs and CVVBs. According to our previous works [42,45], a single metasurface plate can be used to generate CVB with a linearly polarized Gaussian beam incident, and cascading two metasurface plates with a QWP can produce CVVBs, which are shown in parts (ii) and (iii) of Fig.
![(a) and (b) Experimental results of the VBs (the topological charges are 1 and 2) diffracting through the metasurface. (c) and (d) Experimental results of the CVBs (the polarization orders are −1 and 2) diffracting through the metasurface. (e) and (f) Experimental results of the CVVBs [the topological charges and polarization orders are (1, 2) and (2, −1)] diffracting through the metasurface. The gray lines represent the theoretical polarization profiles.](/richHtml/prj/2020/8/6/06000963/img_008.jpg)
Fig. 8. (a) and (b) Experimental results of the VBs (the topological charges are 1 and 2) diffracting through the metasurface. (c) and (d) Experimental results of the CVBs (the polarization orders are and 2) diffracting through the metasurface. (e) and (f) Experimental results of the CVVBs [the topological charges and polarization orders are (1, 2) and (2, )] diffracting through the metasurface. The gray lines represent the theoretical polarization profiles.
Regarding the vertical diffraction pattern and corresponding to the LCP VB, the direction of dark fringes is obliquely downward, indicating that the sign of topological charge is positive. On the contrary, the sign of topological charge is negative. In the horizontal diffraction pattern, the relationship is also valid, and we just need to rotate the diffraction pattern about 90° clockwise. Based on this relationship, the topological charges of LCP () and RCP () components from the VBs are (1, 1) and (2, 2), CVBs are (1, ) and (, 2), and CVVBs are (, 3) and (3, 1). The topological charge () and polarization order () of these singular beams can be calculated by , . Finally, by substituting with (1, 1), (2, 2), (1, ), (, 2), (, 3), and (3, 1), the calculated of singular beams is (1, 0), (2, 0), (0, ) (0, 2), (1, 2), and (2, ), respectively.
4. DISCUSSION
Conventional tunable SDSs are realized via tailoring the structure of devices or the incident light beams, which are not good for integration systems. Except for the SDS, extra phase modulation functions are always pursued for performing more operations on the spin components, which is hard to meet with conventional methods. Tunable metasurfaces based on phase change and elastic materials are potential solutions because the characteristics of materials are thermally and mechanically tunable. It has also been demonstrated that a single metasurface can be used to perform multiple functions by integrating different functional phases. The chalcogenide phase change material composed of alloys of germanium, antimony, and tellurium can be transited between the disordered-amorphous state and ordered-crystalline state with temperature control, which is used for manipulating the “ON” and “OFF” state of the metasurface. However, the manipulation ability in the regulation range and continuous adjustability of these methods are quite limited because of the finite response states of materials. Hence, the controllable SHEs might come from a new tuning mechanism without involving tunable materials. Through mathematical deduction, we found that the phases with the functional structures of , , and adding together gradient phases are equivalent to performing a coordinate translation to original phases. To achieve controllable SHE, we just need to encode specific phases into the P-B phase devices. The proposed phase function construction method is free of the limitations of materials and shows a powerful ability in manipulating the regulation range, continuous adjustability, and stability. The twisting phase and the dynamical phase lens with the functional structures of and satisfy the conditions and can be used to manipulate the photonic SHE. It is found that the interval and the locations of the two separated spin components can be manipulated via shifting the incident beam with respect to the center of the P-B phase device, which provides a route for controllable SDS. With the action of the original phases, the extra phase modulation of the two spin components can also be achieved simultaneously. Except for the twisting phase and the dynamic phase lens, the other phases with similar functional structures are also expected for modulating beams and developing more interesting applications.
Meanwhile, owing to the dispersion-free characteristic originating from the geometric phase [21], the fabricated P-B phase metasurface is broadband in the visible region with high diffraction efficiency. After making full use of the SDS and the extra phase modulation of original phases, some interesting applications are raised. After passing through the metasurface, the two spin components of the incident beam are separated, and their intensity can be measured separately to detect the polarization ellipticity of light beams. Based on the relationship between the two separated spin components’ interval and the incident position shift, a position sensor can also be constructed. Under the modulation of twisting phases, the metasurface also enables detecting the topological charges and polarization orders of arbitrary singular beams, which is a candidate mode demodulator in singular light modulation communication. Hence, the controllable SHE based on phase function construction might bring new opportunities for exploiting the spin and orbital angular momentum of light for optical information processing and communication. Moreover, the proposed controllable SHE scheme can be generalized to other physical systems because of the similar manipulation mechanism, such as electron beams [46].
5. CONCLUSION
In conclusion, we propose a controllable SHE mechanism based on phase function construction, which can be used for manipulating the SDS of light in the momentum space. Moreover, extra phase modulation of the two spin components during the SDS is achieved with the original phases. The twisting phase, which is achieved by engineering spatially variant nanogrooves in the fused silica glass substrate under the P-B phase mechanism, is selected to verify this mechanism. It is experimentally demonstrated that the tunable SDS can be realized by shifting the incident light onto the metasurface in the whole visible region with diffraction efficiency up to 98.72%, 95.05%, and 90.06% at the wavelengths of 633, 532, and 475 nm. The polarization ellipticity detection is also demonstrated with the help of the SDS effect. Furthermore, the extra phase modulation of the twisting phase is successfully used for detecting the topological charge and polarization order of singular beams. It is proved that the proposed controllable SHE based on phase function construction can be used not only for manipulating the photonic SDS but also other applications with the original phase modulation. This controllable SHE manipulation method provides a possible route for manipulating spin-polarized photons and is also promising for optical sensing and communication.
Article Outline
Yanliang He, Zhiqiang Xie, Bo Yang, Xueyu Chen, Junmin Liu, Huapeng Ye, Xinxing Zhou, Ying Li, Shuqing Chen, Dianyuan Fan. Controllable photonic spin Hall effect with phase function construction[J]. Photonics Research, 2020, 8(6): 06000963.