Ion emission from warm dense matter produced by irradiation with a soft x-ray free-electron laser
1 I. INTRODUCTION
Experiments devoted to laser–matter interaction have provided characteristics of various mechanisms of generation and acceleration of ions, which have not only stimulated ideas for many applications, but also contributed to the understanding of laser–matter interactions. Despite the small number of free-electron laser (FEL) x-ray facilities around the world, they are very attractive areas of research such as the science of warm dense matter (WDM) and high energy density,1–3 multiphoton ionization,4 coherent diffraction imaging,5 femtosecond x-ray holography,6 and cluster physics.7 Although many diagnostic techniques have been introduced for the probing of WDM, as presented by Falk,8 comprehensive research on ion emission seems to remain in the background.
The ablation of thick targets by femtosecond laser pulses and the associated ablation rates have been elucidated using models considering the thermal dependences of the optical and thermal parameters of the irradiated materials.9–11 It is evident that ultrashort laser pulses can create a very high temperature within a very small volume of both metal and polymer targets. In some experiments, it is important to show that they provide negligible heat diffusion into the material. In such cases, the profile of the crater created on the surface of the target can be used to characterize focused soft x-ray laser beams.12,13
On the other hand, when plasma is produced by the interaction of a femtosecond x-ray laser with a target, the obtained dependence of ion emission on the focus position can determine the optimal focus position, which has a strong dependence on peak intensity.14–16 In this case, the position of the focal plane refers to the position where the highest proton energy is measured. However, when the crater created does not have a single-hole shape with a smooth surface, as shown in Refs. 12 and 13, but a complex structure of discrete, clearly outlined circular holes and shallow lobes at the bottom of the ablated craters, as shown in Ref. 14, then the secondary craters can become secondary sources of ions expanding into the vacuum with different velocity distributions.
In this paper, we apply a simple model of a signal from time-of-flight (TOF) detectors, which is based on the shifted Maxwell–Boltzmann distributions, to quantify the parameters of ion currents emitted by plasma produced with the use of an FEL. Application of this model makes it possible to reveal partial currents of ionized species, j, which form the total current of ions detected with the use of an ion detector located outside the critical zone, where the charge states of ions of the expanding plasma are frozen.17 This model allows us to determine the temperature Ti and center-of-mass kinetic energy ECM-i of ions. The value of ECM-i indicates whether the ion groups are accelerated by an electrostatic potential (ϕef = ECM-i/e) generated by the release of fast electrons from the plasma (ECM-i > 0) or whether they are only a part of thermal plasma (ECM-i = 0) freely expanding into vacuum.
The remainder of this paper is organized as follows. Section II describes the experimental station at the soft x-ray free-electron laser FLASH2 at DESY, Hamburg and the TOF detector that was used, as well as a method for determining the morphology of the crater surface. Section III presents an analysis of TOF signals and characteristics of ion emission, which are based on a three-dimensional model of the TOF signals detailed in the Appendix. To conclude, we discuss the correlation between the revealed currents of singly charged ions and the complex morphology of the crater surface.
2 II. EXPERIMENTAL ARRANGEMENT
Foil targets of Al, Cu, Fe, Ni, Si, GaAs, and poly(methyl methacrylate) (PMMA), ranging in thickness from 0.1 to 1 mm, were irradiated with the soft x-ray FEL FLASH2 using a Kirkpatrick–Baez focusing system with a focal length of 200 cm to focus 100-fs pulses with energies between 10 and 100 µJ and a wavelength of 13.5 nm (91.8 eV photon energy) to a 36 μm-sized spot surrounded by shallow lobes, resulting in intensities reaching 1013 W/cm2 on targets.18 Since the x-ray attenuation length Latt < 2 µm for the targets used, the target thickness does not affect the laser–target interaction. The pulse energy was obtained from an in-line gas monitor detector (GMD), prior to arrival of the pulses at the interaction region.19 The crater characteristics were obtained with the use of Nomarski-type microscopy. The angle of incidence of the laser beam on the target was 22.5°, as shown in Fig. 1. The fluctuation of the laser energy from pulse to pulse was below 10%. Exposures were done at various focal positions (FP) relative to the target surface to determine the focus position FP = 0, which corresponds to a coincidence of the minimum focus with the front target surface. The convention used is as follows: FP < 0 means the focus position is in front of the target surface and FP > 0 means it falls inside or behind the target. The FP was moved in the range from −20 to 20 mm. The samples were further inspected by Nomarski optical microscopy and white-light interferometry.
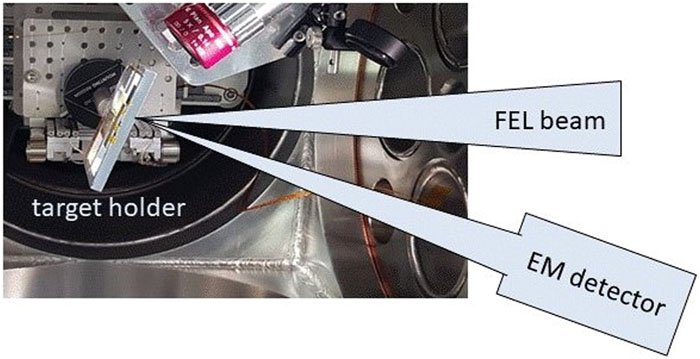
Fig. 1. Experimental setup of the ion experiment at FLASH2. The FEL beam hits the target from the right at a 22.5° angle. Ions are detected along the target normal with an open electron multiplier located 24 cm from the target.
The produced ions were detected with the use of an open electron multiplier (EM) type 119EM along the target surface normal at 24 cm from the target. This windowless electron multiplier equipped with Be–Cu dynodes was manufactured by Thorn EMI Electron Tubes, UK. It has a Venetian blind structure, with a ten-slat entrance dynode with radiation aperture of 2.16 cm diameter and a total of 17 dynodes. The initial amplification of electron current certified by the manufacturer was 6.26 × 106 with an operating voltage of −3 kV applied to the first dynode, while the input grid was grounded. The cross-section of a measured ion beam overlapped the input (radiative) aperture of the 119EM. Calibration of electron multipliers is needed for ion detection, because the ion-induced emission of electrons from the first dynode depends not only on the quality of the dynode’s surface but also on the intercepted ion species and their potential and kinetic energies.20 The calibration has shown that the EM gain depends mainly on the charge state of detected ions, with a weaker dependence on the impact velocity. Since the we were unable to calibrate the gain of the open EM during this experiment, we used previous data21 for the range of low ion kinetic energies encountered in our experiment to determine the value of this gain as 5 × 105.
For the analysis of the EM signal, it is necessary to find out how this signal depends on the velocity, energy, and number of ions incident on the first EM dynode. This dependence is characterized by a parameter α, the value of which affects the shape of the TOF signal according to the three-dimensional model of an ion detector signal recalled in the Appendix:

Fig. 2. Signal of the 119EM electron multiplier induced by ions produced by interaction of FEL beam with Ni target (solid line) and TOF dependence of impact velocity of Niq + ions on the first dynode affected by an EM bias of −3 kV, which was calculated for charge states q of 1–3 (dashed lines).
The fluence profile of the 13.5 eV FEL beam focused on the target and the corresponding spot size were determined using the fluence scan (F-scan) method and the nonlinear response function recovery (NoReFry) algorithm.22,23 Both methods use laser-induced ablation in PMMA to determine the profile of the non-Gaussian energy distribution of the focused laser beam, which can significantly affect the experimental characteristics of ion emission. While the fluence scan method12 exploits microscope images of PMMA ablative imprints created on the target surface by the focused laser beam, the NoReFry algorithm23 works with two-dimensional depth profiles of ablated craters acquired using atomic force microscopy (AFM). The F-scan method recovers beam iso-fluence contours at various fractional levels with respect to the maximum, from which the fluence curve is derived. The NoReFry algorithm retrieves a general two-dimensional fluence profile. The effective areas Aeff of the foci as determined using the F-scan method and NoReFry algorithm are 1027 ± 123 µm2 and 952 ± 247 µm2, respectively. The calibration curve, which determines the dependence of the crater depth on the pulse energy of the 13.5 nm radiation, allowed us to determine the radiation attenuation length Latt = 178 ± 5 nm for the PMMA target that was used. The normalized fluence profile, obtained by converting the AFM-measured imprints on the PMMA target to laser fluence with the use of the calibration curve, is shown in Fig. 3. The reconstruction of the laser energy profile shows two intense peaks surrounded by wide, low-intensity lobes. The black solid curve drawn in the iso-smooth contour (see Fig. 3, right) encloses an area equal to an effective area of 1027 µm2, corresponding to an effective beam radius of 36 µm.

Fig. 3. Normalized fluence profile of the focused laser beam obtained by converting the measured imprints, i.e., the depth profile of the crater created on the surface of the PMMA target, to laser fluence. The black solid curve indicates an iso-fluence contour encircling an area equal to the effective area of 1027 µ m2, which corresponds to an effective beam radius of 36 µ m.
3 III. EXPERIMENTAL RESULTS AND DISCUSSION
3.1 A. Ion sound velocity
The TOF spectra of ions produced by focusing the soft x-ray FLASH2 beam on thick Al, Fe, Ni, GaAs, PMMA, and Si foil targets had to be detected with the use of an electron multiplier because of their very small current at the nanoampere level. Figure 4 shows typical TOF spectra of ions emitted by irradiated targets. These spectra show similar behavior in each material, since they have two dominant maxima corresponding to fast and slow ions. Thus, we can assume that the TOF spectrum consists of a series of individual, overlapping currents of various ionized species. The fastest ions, which form the front of the expanding plasma plume, are hydrogen ions produced from chemisorbed impurities, such as hydrocarbons, on the target surface.14,15 These protons should be accompanied by carbon and eventually oxygen ions that again have been produced from impurities. In addition to these ionized impurities, the TOF spectrum may also contain currents of slowly propagating charged clusters of the heavier target atoms.14
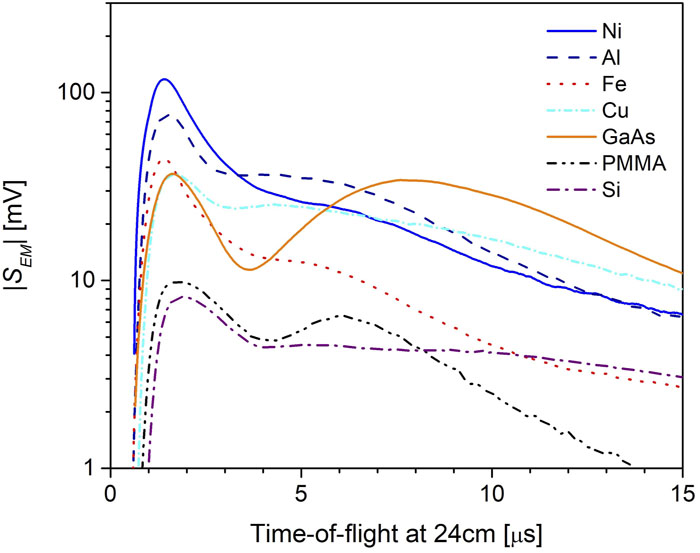
Fig. 4. Signals from the 119EM electron multiplier induced by ions produced by interaction of the soft x-ray FLASH2 beam with Al, Fe, GaAs, Ni, PMMA, and Si targets. The TOF spectra were measured at 24 cm from the targets. The intensity on the targets was 2.8 × 1013 W/cm2 and FP = 0.
Separation of the laser-produced plasma into fast and slow groups is a common phenomenon observed in other experiments performed in various laboratories using both FEL and standard femtosecond lasers.14,24–27 Another way to demonstrate the separation of fast and slow ions is to transform the ion detector signal into the spatial distribution of the ion charge density Qi at a selected time τ that has elapsed since the laser–target interaction. This is made possible by a method based on transformation of the TOF signal acquired by the detector into a DOF (distance-of-flight) dependence of the ion charge density Qi(z, τ). This method was derived and experimentally verified for ions that have passed a critical distance beyond which recombination of ions is negligible; in this case, the charges are non-interacting and thus the ion charges are “frozen”. The similarity relation for the ion current density measured at different distances from the target is j(z, τ)z3 = j(L, t)L3, where z/τ = L/t = v, which follows from the assumption of conservation of the charge carried by ions far from the target, whose density decreases as L−2. This can be also derived from Eq. (1) by modifying the term L/t4 to v4/L3 in , where dS is the area element of the ion detector, as provided in the Appendix. As a result, the detector signal decreases with distance as L−3. The TOF t is transformed to the DOF z using the relationship z = Lτ/t, where L is the detector distance from the target. The values of τ and L are kept fixed. On substituting t by z, the ion current density j(L, t) = Q(L, t) v can be transformed to the space-resolved ion charge density at τ as28
DOF spectra are presented in Fig. 5 for τ = 1 µs. These spectra also show how far from the target the ion front has spread within 1 µs. Since the ion expansion is closely related to the electron distribution, the ion density should decrease exponentially with distance. This can be expressed in the form ni(z, t) = n0 exp(−v/cs), where ni(z, t) is the density of those ions traveling at velocity v, n0 is the density at the emitting surface, and cs is the ion sound velocity.29 Substituting v = z/τ gives
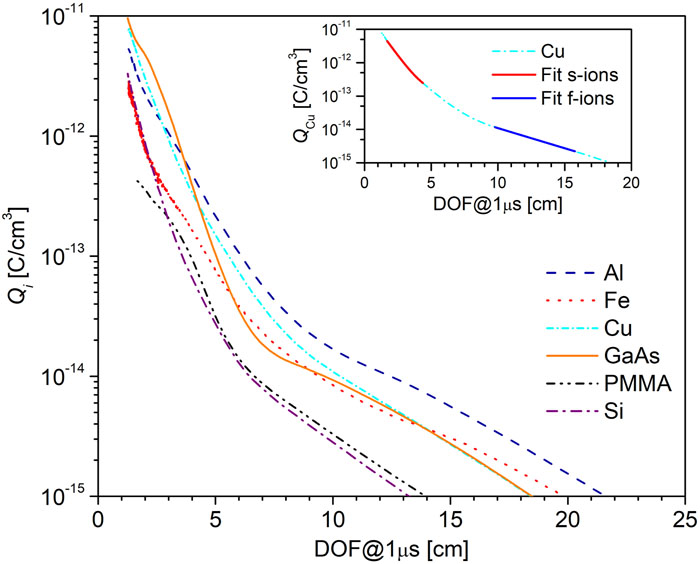
Fig. 5. DOF spectra obtained by transformation of signals from the 119EM electron multiplier induced by ions produced by interaction of the soft x-ray FLASH2 beam with Al, Fe, GaAs, Ni, PMMA, and Si targets for a time of 1 µ s that has elapsed since the laser–target interaction. The intensity on the targets was 2.8 × 1013 W/cm2. The inset shows two fits of Eq. (3) to the DOF spectrum of Cu ions to estimate the ion sound velocity associated with slow (red line) and fast (blue line) ions.
A fit of the exponentially decreasing function to the DOF spectrum of Cu ions shown in the inset of Fig. 5 gives kTef values of ≈40 and ≈840 eV. These values can be interpreted as the kTef corresponding to slow and fast ions.30 In summary, the analysis of the DOF spectra of all studied elements using Eq. (3) shows that kTef of fast ions reaches hundreds of eV (i.e., 200–1000 eV), while for slow ions it is tens of eV (10–90 eV).
The two-temperature model still does not fit the data exactly, and therefore we use a multitemperature model with partial currents. Ions are not only separated into two basic groups of fast and slow ions, but into several groups with different expansion velocities. In addition to temperature, the expansion velocity of ions is also affected by the center-of-mass velocity that the ions acquire by accelerating in an electrostatic field created by escaping fast electrons, as will be discussed below. This fact should also be reflected in the parameters of partial currents revealed by decomposition of TOF spectra. A break in the exponential decrease in density with increasing distance from the target should also occur at the front of the plasma, which contains fast hydrogen ions, i.e., the lightest species. However, owing to the high velocity of H+ ions, this break should occur at DOF ≈ 20−50 cm for τ = 1 µs, where their density is quite negligible. The decline in the rate of decrease of charge density, located at a distance of 5–10 cm from the target, corresponds to the minima of the TOF spectra, occurring at 3–5 µs after the pulse (see Fig. 4). In this location, no hydrogen impurities can occur, and hence the rate drop can be interpreted as a significant change in temperature of Cu ions.
3.2 B. Ion velocity distribution
Since the use of an ion mass analyzer was not possible in this experiment, the number of partial ion currents could only be estimated by fitting the TOF spectra with a composite function S consisting of variable number of currents. For revealing these partial currents, we used a signal function recalled in the Appendix, which is based on the shifted Maxwell–Boltzmann distribution:
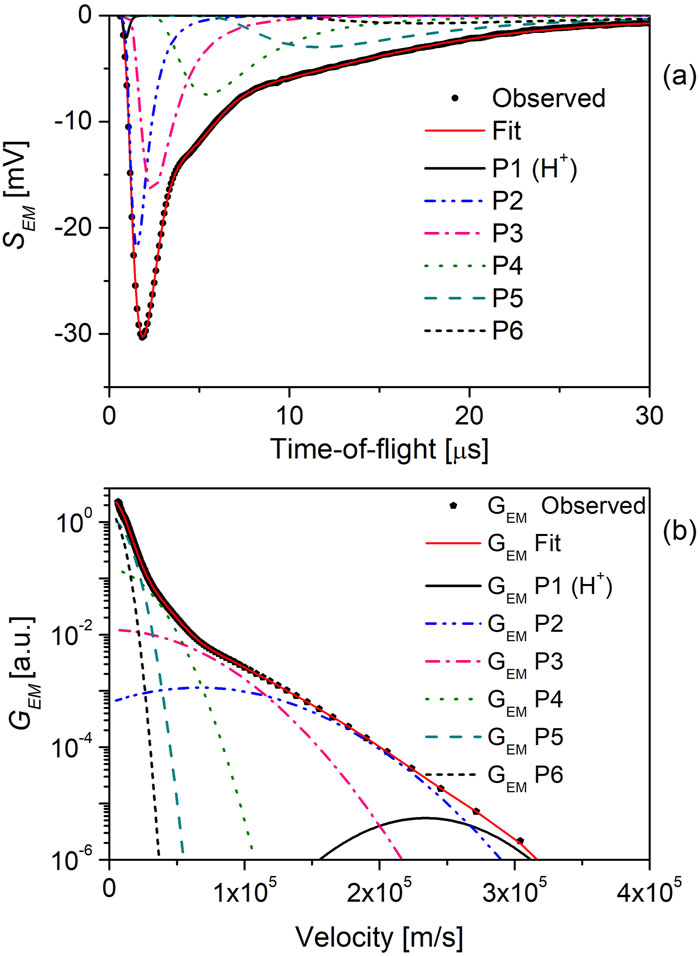
Fig. 6. (a) Fitting of TOF spectrum of ions to partial currents P1–P6. (b) Gaussian parts G EM of P1–P6 currents of the TOF signal function in (a). P1 and P2 peaks of beam-like ions (u CM-P 1 ≈ 2.3 × 105 and u CM-P 2 ≈ 6.7 × 104 m/s) and P3, …, P6 peaks of thermal ions emitted by Ni target irradiated with 1.4 × 1013 W/cm2.
Figure 6(b) shows the Gaussian part of the decomposed detector signal defined as
Along with the ionized target species, ionized surface impurities are also emitted from the irradiated target surface.14,15 A typical contaminant is hydrogen, whose ions emitted from the periphery of the crater can expand at a velocity comparable to that of the slower ions forming the group of fast ions, and therefore can cause signal smearing. However, their contribution to signal smearing depends on their amount, which tends to be negligible compared with the amount of ionized target species. Thus, although a very small number of slow H+ ions can be detected using a mass analyzer, the fitting (4) to the detector signal may not identify them because, for example, the second derivative of the detector signal that should indicate them does not show a clear change.31
Fitting of Eq. (4) to the ion current emitted from the irradiated Ni target reveals six groups of ions. Consistent with other experiments, the fastest group, labelled P1, can be considered as a group of fast H+ ions.14,31 These ions are accelerated to the highest velocity and move at the front of the expanding plasma. The following peaks should correspond to multiply charged ions of the irradiated target elements. In a plasma with several ion species, each with a different value of q/mi, the ambipolar electric field that appears in the collisional regime tends to accelerate these different species relative to each other.32 This leads to their observable separation during expansion, where their charge gradually decreases with decreasing expansion velocity.33,34
Figure 6(b) shows two ion groups P1 and P2, which consist of beam-like ions accelerated by escaped electrons, because their Gaussian distribution has a maximum at velocities of 2.3 × 105 m/s for hydrogen ions and 6.7 × 105 m/s for Ni ions. The potential corresponding to the acceleration of H+ ions was about 300 V. This indicates that in the plasma front layer there are fast electrons escaping from the plasma and a potential barrier accelerating the ions. This process is influenced by collisions of electrons before their escape, the ratio of the height of the potential barrier to the temperature of fast electrons, oscillations of the potential barrier, etc. This potential partially shielded by H+ ions also accelerated the P2 group of Ni+ ions to a center-of-mass energy of 1.3 keV. This allows us to estimate the charge state of these ions to be 5. The charge state of the slower groups should gradually decrease to 1, as mentioned above. Conversely, the other four ion groups have Gaussian distributions GEMP3, …, GEMP6 centered around zero because their uCM ≈ 0. Therefore, they can be considered as thermal ions. The kT parameters of the P1 and P2 currents reached values of about 20 eV and 2.2 keV, respectively, while the slower thermal ions forming the P3–P6 groups reached lower values of about 1.5 keV, 300, 65, and 30 eV, respectively.
Numerical simulations of the plasma produced on bulk samples, for example, of niobium and vanadium, by the FLASH laser have shown that the plasma reaches temperatures between 100 and 400 eV at a power density above 1016 W/cm2.14 Although the intensity in our experiment was three orders of magnitude lower, the observed temperature of the fastest ion groups was comparable or higher, as shown in Fig. 7, where the temperature is related to the relative atomic mass Ar. [The term mi/kT in Eq. (4) is substituted by mu/(kT/Ar), where mu is the atomic mass constant.] The temperature of the fastest ion group (labelled as P1) can be interpreted as the temperature of H+ ions in all TOF spectra.

Fig. 7. Temperature per relative atomic mass kT /A r for irradiated targets vs order of ion groups (partial peaks). Numbering of the revealed ion groups starts from the fastest one.
The best fits showed that all ion currents except the Si ion current consisted of six partial currents, while for Si ions seven partial currents were revealed. The seventh group represents the slowest ions forming the long tail of the TOF spectrum, which is not found in other plasmas, as shown in Fig. 4. Some experiments have shown that there are groups of the slowest singly charged ions forming very long tails of TOF spectra, which can be interpreted as the sum of two groups.33 Therefore, both groups P6 and P7 could be formed only by Si+ ions.
As shown in Fig. 7, kT/Ar values of Cu ions (P2–P6 peaks) decrease with the peak number almost exponentially from ∼26 to 0.4. Comparison of the relevant values of kT ≈ 1600 and 30 eV with the kTef ≈ 840 and 40 eV evaluated using the ion sound model (3) indicates (i) Tef represents the average temperature of corresponding ion groups, (ii) the contribution of Te to the ion sound velocity cs is negligible.
The temperature per relative atomic mass kT/Ar of the first two ion groups of the Si plasma shown in Fig. 7 reaches the highest values of all five irradiated target materials, even though their total current is the lowest. It is evident that the decrease in temperature of H+ ions is influenced by the properties of the target, such as electrical conductivity, which affects the energy distribution of laser-excited electrons between chemisorbed and target atoms. Figure 7 helps to recognize the gradual temperature decrease from Si to Al and Cu targets, as the electrical conductivity of Al and Cu increases. However, Ni and Fe targets show the lowest temperature of H+ ions, indicating that the H+ ions have not been thermalized, because they gained center-of-mass energy. Conversely, all groups of Si, Al, and Cu ions have uCM ≈ 0 and are therefore thermal ions. The slower groups of Ni and Fe ions, i.e., groups 3, 4, etc., also have uCM ≈ 0. Compared with the properties of ions produced by IR–UV lasers, this is an exceptional case, because usually all ion groups have a nonzero uCM.34 This result could indicate that the value of the applied fluence is close to the threshold at which enough fast electrons are produced capable of contributing to the acceleration of Ni and Fe ions expanding into the vacuum, at the expense of their complete thermalization. It can be expected that a similar phenomenon would occur with other materials if the device used could provide more energy. It is interesting that of all the materials used, it is the Ni and Fe targets that emit the largest number of fast ions from the total number of produced ions. This could also be related to the morphology of the craters, which varies from one material to another in a strong dependence on the properties of the laser pulse, specifically on their periphery which is the source of slow ions. This fact would indicate that chemical bonds of impurities on the target surface, defects in the surface, and the amount of disorder usually present on surfaces also affect the energy distribution of excited electrons. Thus, the results need further theoretical and experimental investigation to be fully understood.
The formation of slower groups of ions may be related to a secondary process triggered by an imperfection of the laser beam, which can be determined from the crater morphology. In our case, there would have to be several secondary ion sources, possibly as many as seven, as can be inferred from the number of sub-craters shown in Fig. 8 for the Si and Cu targets. The observed crater morphology follows the power density distribution in the beam profile, which is affected by astigmatism and other aberrations of the laser beam originating predominantly from the beamline optics and the undulator source. The created sub-craters can emit ions because the resolidification of the surface layer takes about 100 ns.35

Fig. 8. Ablative imprints in (a) Si and (b) Cu created by 13.5-nm FLASH2 beam delivering energies of 100 and 50 µ J, respectively, on the targets.
In an ideal experiment, the crater formed around the circular focus acts as the sole source of ions. However, the high number of ion groups with uCM ≈ 0 indicates that the crater formed on the Si target, as Fig. 8(a) shows, can be understood as a complex of at least four sub-craters whose morphology corresponds to the power density distribution in the laser beam profile. This can provide an explanation for the observed multiple temperature phenomena. Figure 8(b) shows the threshold contour surrounding two circular craters localized at the intensity maxima of the laser beam (see Fig. 3) and an imprint of the peripheral surroundings of the central laser beam on the front surface of the Cu target. This beam imprint is larger than the imprint on the Si target, although the energy supplied was halved, i.e., 50 µJ, which indicates that the ablation threshold of Cu is lower than of Si at the wavelength of 13.5 nm.
The difference in the morphology of the craters on Si and Cu target surfaces may be due to different material properties, such as the attenuation length Latt of the FEL radiation in the surface layer, where the Latt in Cu is about 40 times shorter than that in Si,36 as well as different material responses at applied FEL intensities,37 the surface roughness height, etc. The absorption length is also related to the ablation threshold. More-penetrating radiation usually has a higher ablation threshold in terms of threshold fluence. Since the FEL (92 eV) is tuned close to the L-edge of silicon (99 eV), the radiation absorption is very low. In conclusion, the complex structure of discrete, clearly outlined circular/oval holes and shallow lobes at the bottom of ablated craters can act as a series of almost independent ion sources producing mostly thermal ions with various temperatures. Thus, the morphology of the crater surface can be interpreted as an assemblage of several separate sub-craters that reflect the fluence profile of the focused laser beam and that act as separate ion sources. This fact could be detected thanks to the imperfect profile of the laser beam.
In contrast to the presented single-element materials, the separation of GaAs plasma into fast and slow groups is the largest. However, owing to the mass proximity of Ga and As ions, there is no significant spatiotemporal separation between the expanding 31Ga and 33As ions, for both slow and fast ions. This proximity does not allow the currents of Ga and As ions to be distinguished from each other. The sum of their currents can be interpreted as a current of ions with an atomic mass equal to the average mass of the participating elements.
The fast bunch of GaAs plasma expands into vacuum at a peak velocity of 1.5 × 105 m/s, which is 4.75 times the expansion velocity of the slow plasma. The fast plasma manifests as a pair of partial peaks P1 and P2, where the peak P1 corresponds to beam-like H+ ions because their ECM ≈ 25 eV and kT ≈ 80 eV. The second peak P2 is composed only of thermal ions with kT/Ar ≈ 49 eV and ECM ≈ 0. The slow thermal ions forming peaks P3–P5 have kT/Ar < 3 eV. This is the real reason for their separation during their expansion into the vacuum and the corresponding discontinuity in the exponential decrease in the DOF spectrum of the ion charge density. Since the Fig. 9 shows that the highest energy density supplied by the FEL onto the target surface was concentrated in two paraboloidal craters, we can assume that the fast plasma, i.e., the P1 and P2 peaks, originates from this pair of craters, while the slow plasma forming the P3–P5 groups of thermal ions comes from the shallow lobes. The deep minimum in the TOF spectrum [see Fig. 9(b)] between the groups of peaks P1–P2 and P3–P5 proves that there is a difference between their acceleration mechanisms.
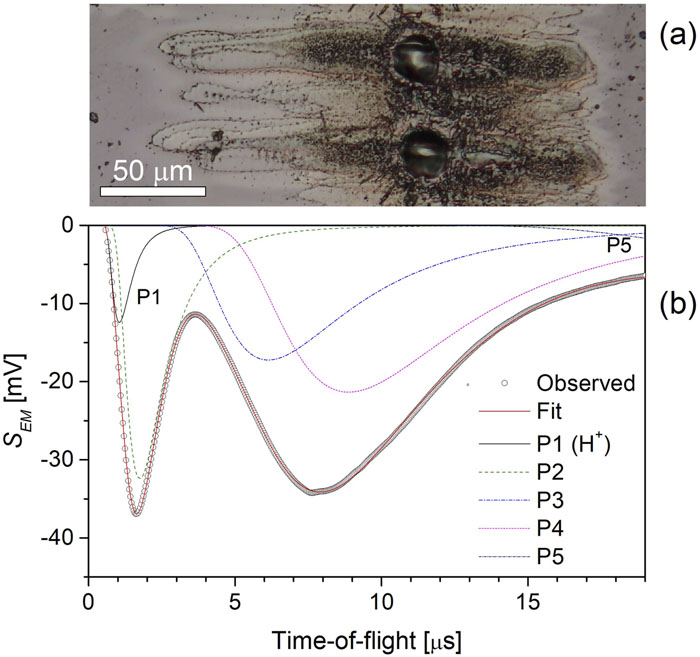
Fig. 9. (a) Ablative imprints in GaAs and (b) TOF spectrum of ions emitted by the GaAs target irradiated with energy 100 µ J (2.7 × 1013 W/cm2).
As an example, Fig. 10 shows the laser energy dependence of the peak energy Epeak/Ar of partial currents of expanding Ni plasma. The numbering of the partial current is related to the highest energy Epeak/Ar, where the fastest group of H+ ions is labelled P1. The novelty of this experiment is that increasing the energy from 10 to 50 µJ creates a new fast ion peak, while the number of slow partial ion groups is preserved. This new peak (P2) could be interpreted as a group of Ni5+ ions, while the highest charge state of the Ni+ ions produced at 10 µJ is 4 (here, the group of Ni4+ ions is also labelled P2). A further increase in laser energy only increases the magnitude of the current and the temperature of the ions, while the number of ion groups does not change. Since the ion current and the ion expansion velocity increase with increasing laser energy, the dependence of the Ni ion emission on laser energy does not bring any unexpected changes.
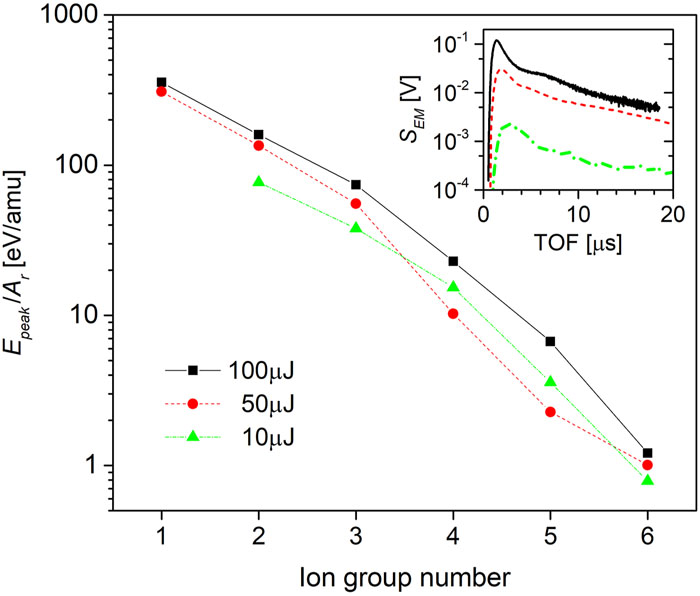
Fig. 10. Peak energy per relative atomic mass E peak/A r of partial ion groups revealed from TOF spectra. E peak corresponds to the peak current of ions forming the n th group. The inset shows the corresponding TOF spectra of ions produced on a Ni target exposed to laser energies of 100, 50, and 10 µ J.
3.3 C. Energy and charge carried by ions
To gain a more comprehensive understanding of the production of fast and slow ions, we will consider the energy EIC of the ion collector signal as represented by the output voltage UIC that is induced by the current of the captured ions:
Applying this method, we can determine the amount of laser pulse energy that has been transferred by ions to the ion collector circuit, as shown in Fig. 11. Evident separation of fast ions from slow ions makes it easy to identify their gradual contribution to energy transfer. In the first period after laser interaction with a target, lasting 4 μs, only fast ions transfer energy into the electrical circuit of the ion detector. Then, the fast ions are replaced by slow ions, which transfer energy for another 15 µs. Figure 11 shows that from the laser energy of 100 µJ, only 7 × 10−22 J was transferred into the ion collector circuit. Thus, the efficiency of this energy transfer is only ≈7 × 10−18. The contribution of slow ions to the energy transfer is ≈3.8 times higher than that of fast ions, as estimated from the partial ion currents revealed in Fig. 10. We note that the group of fast ions consists of partial currents of P1 and P2 groups, and the partial currents P3–P5 form the current of slow ions.
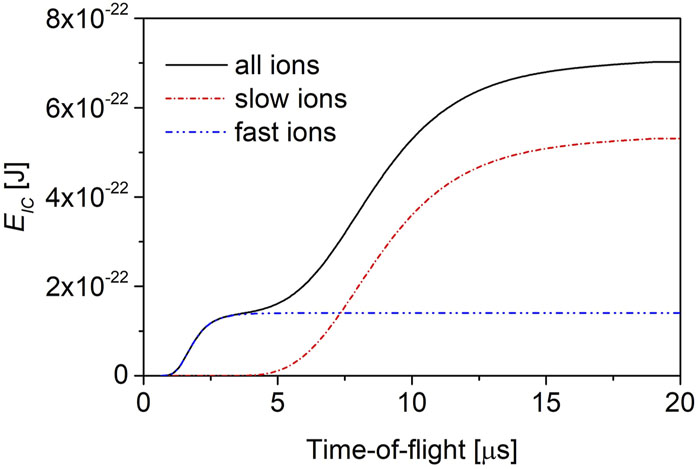
Fig. 11. Calculated time course of the energy E IC transferred by current j IC flowing through the input impedance of the oscilloscope. The solid line is for “all ions,” for which j IC = S EM/1.25 × 1013 A, where S EM is the EM signal (see Fig. 9 ). The other two lines show the hypothetical cases of generation of either fast or slow ions by a GaAs target irradiated with the 13.5-nm FLASH2 FEL.
In general, H+ ions followed by target ions having the highest kinetic energy and charge state form the front of the expanding plasma.33,34 Since the ion mass analyzer could not be used in this experiment, the highest charge q state of the dominant ionized species can only be estimated. At lower intensities of the FLASH2 FEL, multiple charged ions were observed in Refs. 14–16. In our experiment, the highest value of q could reach 5, depending on the target element, as already experimentally demonstrated for the same photon energy in Ref. 16. Recombination of ions gradually reduces q as they become neutralized. In addition, ionized clusters and neutral particles can be produced. Because the electrostatic field accelerating the ions was very small in our experiment and affected mainly ionized hydrogen (see Figs. 5 and 6), there was no force to visibly separate the ions with different charges as observed in other experiments.33,34 The presented decomposition of the TOF spectra using the Maxwell–Boltzmann distribution shows that the ions were separated according to their temperature. This separation of ions by temperature, but without the presence of a field, does not mean that the revealed partial currents are composed only of ions having the same charge and mass. In the case of PMMA, the current of slow ions coming from the shallow lobes of the craters exposed to low intensity can be composed of ionized fragments, for example, C2H3+, COH+ and/or C2H5+, C3H3+, COCH+ and/or C3H5+, COCH3+ and/or C3H7+, COOC+ and COOCH+, as observed by photon-stimulated desorption of ions from a solid PMMA target irradiated with synchrotron radiation.38
A specific feature of our experiment is the very short attenuation length of the FLASH2 radiation in irradiated target materials, which ranges from tens to hundreds of nanometers. By irradiating the surface of different materials with the same laser intensity, we can obtain the dependence of the charge carried by ions (i.e., the time integral of the observed ion current) on the attenuation length Latt, as shown in Fig. 12 for optimal focusing (FP = 0). We note that the attenuation length was not corrected for the angle of incidence in this case. The values of the penetration depth indicate that the laser intensity is absorbed in the first atomic layers, leading to ablation and plasma production in the upper surface layer. The increasing Latt leads to a decrease in the deposited energy density heating the irradiated volume, which causes a decrease in the energy efficiency of the laser in ion production. The experiment apparently shows that less energy is stored in the sharp circular focus than outside because the fast ions carry less charge than the slow ions from the shallow lobes of the crater.
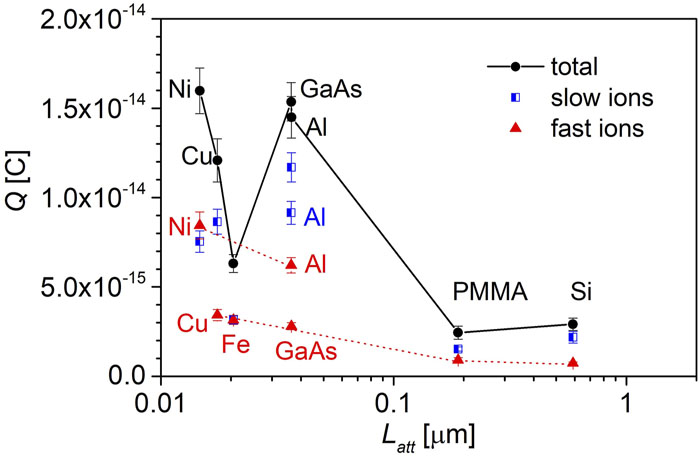
Fig. 12. Dependence of the charges Q fast and Q slow carried by fast and slow ions, respectively, and their sum on the attenuation length of 13.5 nm laser radiation in different targets. Attenuation lengths were calculated for all the target materials used using the Henke method.36
Determining the magnitude of the charges carried by the revealed currents shows that ion emission is affected by various interactions between laser-accelerated electrons with surface and lattice atoms. In the case of fast ions, the charge decreases with increasing penetration length of the 13.5 nm radiation. However, the charge of Ni and Al ions is about 2.5 times higher than that of the other fast ions. Higher charge yield is shown by slow ions. There are also two groups of material that differ in charge yield. The charge of slow ions emitted from Ni, Cu, Al, and GaAs materials is about four times greater than that from Fe, PMMA, and Si targets.
Since the laser intensity is more than ten times lower on the crater periphery than in the crater center (see the “effective area” in Fig. 3) and the charge yield is higher from the peripheral surroundings, the similarity in ion charge yields related to laser intensity or fluence is not relevant here. Thus, it can be assumed that the area of the crater periphery and post-interaction surface processes control the emission of slow ions whose temperature per relative atomic mass kT/Ar ≲ 10 eV, as shown in Fig. 7, and the charge state of ionized fragments is low, mainly 1. The charges of Ni, Fe, PMMA, and Al fast ions are comparable to the charge of slow ions, while the charges of GaAs, Cu, and Si slow ions are about 3.5 times higher than that of fast ions. Other phenomena such as rapid surface pyrolysis of GaAs and other thermodynamic properties of GaAs39 also contribute to the complexity of surface processes. It is evident that relaxation processes such as heat transfer from irradiated volume to target during the ablation period also play an important role.
The peculiarity of this experiment is that the ion emission originates from the surface layer, the submicrometer thickness of which depends on the attenuation length of the 13.5 nm FEL FLASH2 radiation in the irradiated targets. Although the results presented here require further theoretical and experimental investigation for their full understanding, it is possible to estimate the differences between the properties of plasmas produced using 100 fs FLASH and 23 ns KrF lasers at comparable fluences of 10–30 J/cm2.28,31,33 The fundamental difference between these experiments lies in the different mechanisms of energy dissipation in the irradiated targets. Whereas the nanosecond laser pulse interacts with the ablated material that remains in contact with the target, the femtosecond laser pulse does not interact with the ablated plasma, because the energy transfer time of the electron gas excited by the femtosecond laser to the lattice through collisions and the initiation of material ablation is about 10 ps. The interaction of the 23 ns KrF laser with the ablated plasma results in acceleration of ions, mainly by escaping electrons,28,32 while the expansion of the ions produced by the FLASH2 laser is primary determined by the temperature of the ion component. Accordingly, the temperature of the ions produced by the soft x-ray laser is two orders of magnitude higher than that of the ions generated by the UV nanosecond laser. Conversely, nanosecond UV ablation of bulk targets produces ∼107 times more ions than femtosecond soft x-ray ablation.
By contrast, a comparison of our extreme ultraviolet (XUV) experiment with an experiment devoted to the ablation of ionized silicon with 160 fs pulses delivered by a Ti:sapphire laser (800 nm, 600 µJ)40 shows that the maximum kinetic energy of ions produced with the XUV laser is about 20 times higher. Numerous other authors41–43 working with ultra-short pulses of UV–Vis–IR laser radiation obtained similar results as Roeterdink’s group.40,44 The basic characteristics of ions and electrons produced and accelerated by UV–Vis–IR lasers are scaled using the similarity parameter Iλ2 in W cm−2µm2.45,46 To achieve a comparable value of maximum ion velocity, the Ti:sapphire laser experiment needs about 10 000 times higher Iλ2, mainly because of the very high value of the square of their wavelength ratio of ∼3500. It is obvious that the properties of ions produced by these lasers cannot be compared using this similarity parameter, and therefore the model of interaction of UV–NIR lasers with matter is not applicable to XUV laser interaction experiments.
Changing the wavelength from 248–800 to 13.5 nm not only reduces the value of Iλ2 by a factor of ∼300 to 3500, respectively, but also results in direct ionization by XUV photons whose energy is above the first ionization potentials of the target atoms. Each of these events also releases an energetic photoelectron. A portion of the photoelectrons can escape from the target into vacuum, carrying with them a cloud of secondary electrons, which are photoelectrons released from the basic atoms or ions of the solid target by collisional ionization. As result, a cloud of electrons is formed above the target surface, while there is a high excess of positive charge in the near-surface layer of the target, and the constitutional ions begin to be ejected from the material by Coulomb repulsion to be further accelerated after ejection by the electric field associated with the cloud of electrons previously released into the vacuum above the surface of the target. Other photoelectrons, however, transfer XUV energy to the target lattice by collisions with subsequent formation of multiply charged ions,15 which contributes to material ablation. Our analysis also shows that the velocity of the fastest ions may contain a component that corresponds to the center-of-mass velocity caused by hot electrons escaping from the plasma, which were captured by the ions and formed a double layer with them.
Although little experimental data have been published to date, and it is therefore not possible to fully characterize XUV ion production, our analysis suggests that direct ionization of target atoms by high-energy XUV photons represents the primary process that distinguishes experiments with XUV lasers from those with UV–Vis–IR lasers.
4 IV. CONCLUSIONS
Ablation of solid films by ultrashort x-ray pulses delivered by the FLASH2 laser leads to the emission of multiple charged ions and ionized clusters. The proportion of both components can be influenced by changing the irradiation conditions and the target material. These conditions are affected by the aberrations of the laser beam and the attenuation length of the delivered 91.8 eV (13.5 nm) photons in targets, which determines the depth of the heated volume, where WDM can be transiently generated at ion temperatures of ≈1 eV.47 Because the number of charged species produced in our experiments was very small, an open electron multiplier with a gain of 5 × 105 had to be used to detect them. We observed that the emission of ions by single-element materials is intense when the penetration depth of radiation is around 10 nm. The plasma expanding into vacuum consists of two ion parts: slow and fast ions, which vary greatly in temperature. The expansion of fast ions, including ionized hydrogen chemisorbed on the target surface, is influenced by the acceleration potential produced by fast electrons escaping from the plasma. Unlike fast ions, slow ions carry a substantial portion of the charge generated by the laser pulse and transfer more energy to the ion collector circuit. We have estimated that the slow ions forming a substantial part of the plasma were emitted by the shallow lobes of the crater formed by the aberrations of the laser beam. Finally, a comparison of two different methods that allow determination of the ion sound velocity and the parameters of partial ion currents have allowed us to show that it is primarily the ion temperature that determines the ion sound velocity.
The transfer of laser energy by excited electrons to the submicrometer surface layer of the target depends not only on the target material, but also on the chemical bonds of contaminants on the target surface, defects in the surface, and the amount of disorder usually present on the surface. It is therefore difficult to draw undisputable conclusions from experiments in which the production of hot electrons and the transport of their energy to surface atoms varies from one target to another.
5 ACKNOWLEDGMENTS
Acknowledgment. This work is partly supported by EURATOM within the “Keep-in-Touch” activities and the Aquitaine Regional Council. We acknowledge financial support from the French National Research Agency (ANR) in the frame of the “Investments for the Future” Programme IdEx Bordeaux-LAPHIA (Grant No. ANR-10-IDEX-03-02), and the Czech Science Foundation (Grant Nos. GM23-05027M and 20-08452S). The team of K.F. are funded by the Helmholtz Association under Grant No. VH-NG-1338. A.F., T.G., T.C., S.R., Y.S., and S.M.V. acknowledge support from UK EPSRC Grant Nos. EP/P015794/1 and EP/W010097/1, and from the Royal Society. J.K., L.J., M.K., T.B., Š.J., and S.K.S. acknowledge support from the Czech Ministry of Education, Youth and Sports (Grant No. LM2023068).
Article Outline
Josef Krása, Tomáš Burian, Věra Hájková, Jaromír Chalupský, Šimon Jelínek, Kateřina Frantálová, Michal Krupka, Zuzana Kuglerová, Sushil Kumar Singh, Vojtěch Vozda, Luděk Vyšín, Michal Šmíd, Pablo Perez-Martin, Marion Kühlman, Juan Pintor, Jakub Cikhardt, Matthias Dreimann, Dennis Eckermann, Felix Rosenthal, Sam M. Vinko, Alessandro Forte, Thomas Gawne, Thomas Campbell, Shenyuan Ren, YuanFeng Shi, Trevor Hutchinson, Oliver Humphries, Thomas Preston, Mikako Makita, Motoaki Nakatsutsumi, Xiayun Pan, Alexander Köhler, Marion Harmand, Sven Toleikis, Katerina Falk, Libor Juha. Ion emission from warm dense matter produced by irradiation with a soft x-ray free-electron laser[J]. Matter and Radiation at Extremes, 2024, 9(1): 016602.