Pulse shape of ultrashort intense laser reflected from a plasma mirror
Download: 1010次
With the increase of achievable intensity of high-power lasers, the requirement of higher temporal contrast, defined as the intensity ratio of the leading front to the main peak, has been a growing issue in laser–plasma interaction experiments[1,2]. Nanosecond-duration amplified spontaneous emission (ASE) and shorter-duration prepulses are sources of the background noise. The latter could be attributed to the nature of non-Gaussian-distributed optical spectrum and the residual spectral chirp or non-ideal optics in the laser chain. As a robust technique, the plasma mirror (PM) has been routinely used to improve the laser temporal contrast. The initially transparent PM substrate is employed to transmit the ASE and prepulses and to reflect the main pulse when the substrate is ionized to the plasma state. A number of experiments and theoretical work have been performed to understand dynamics of the PM and to assess its performances[35" target="_self" style="display: inline;">–
PM-enhanced higher contrast lasers are superior in laser-driven ion acceleration and have the possibility to explore novel acceleration mechanisms with an ultrathin target, i.e., radiation pressure acceleration (RPA)[8,9], breakout afterburner (BOA)[10]. Recent researches show that multiple mechanisms may dominate over time due to intra-pulse intensity temporal evolution[11,12]. The prepulse-free lasers, with an additional laser pulse serving as a controllable prepulse, offer the opportunity for controlling and optimizing higher-order harmonic generation (HHG)[2,13]. The sensitive dependence of ion acceleration and HHG on the exact laser pulse shape poses severe requirements on well characterization and control of the complete laser temporal profiles. Two theoretical works suggested that the PM could steepen the femtosecond rising front for an ideal (Gaussian-distributed) input laser pulse[6,14]. However, the temporal profile of the laser pulse reflected from the PM in the sub-picosecond regime and its sensitivity to experimental conditions were scarcely investigated experimentally so far[6]. The optimized PM performance was operationally chosen based on measurements of the integrated reflectivity and evaluations of the far-field beam qualities by varying the laser fluences on the PM surface[5]. The pulse duration after employing the PM was thought to be unchanged in analyzing experimental results.
In this Letter, we show that the temporal shape of the laser pulse is sensitively influenced by laser fluence on the PM surface. A cleaner pulse can be produced using smaller laser fluence at the expense of reflectivity. The role of plasma formation close to the main pulse on the pulse shape is discussed. The results will extend our knowledge on proper utility of the PM technique for temporal contrast improvement and applications.
This experiment was carried out using the high-power Ti:sapphire laser at Shanghai Jiao Tong University. The lasers could deliver pulses with energy up to 5 J in 10 Hz and pulse duration of

Fig. 1. (a) Schematic of the experimental setup. Two identical off-axis parabola mirrors, OAP1 and OAP2, are used to focus and recollimate the laser beam. The laser pulse temporal profiles are measured with SHG-FROG and Sequoia. W1, W2 are wedged fused silica plates, and M1–M4 are HR mirrors. M2 is mounted on a motorized linear stage. PM stands for plasma mirror. (b) The laser contrast measurement in a 60 ps window using Sequoia. The inset is the zoom-in profile from to 0.5 ps. The ‘a’ and ‘b’ correspond to the estimated PM trigger times for laser fluences of and , respectively. Example results of (c) retrieved FROG trace and (d) the corresponding Wigner distribution of laser pulse without a PM.
The reflected beam from the PM system is sampled by two 20-mm-diameter pick-up mirrors (M3 and M4) for pulse temporal profile measurements. In the sub-picosecond regime, it is single-shot measured using a frequency-resolved optical gating (FROG, GRENOUILLE, Swamp Optics, LLC.) device. The device is based on second harmonic generation (SHG), having 1.2 fs temporal and 1.3 nm spectral resolutions, respectively. The temporal window is 300 fs. Wedged plates and a 1-mm-thick vacuum window both made of fused silica are used to reduce the laser energy into individual diagnostics and minimize the transmission-induced dispersion and B integral[16]. Due to the time direction ambiguity of the autocorrelation process, two possible pulses for each FROG trace could be retrieved. By positively chirping the pulse via inserting a thick glass plate into the beam path, the leading and falling sides could be distinguished[17]. The temporal profile, i.e., intensity contrast, in the sub-nanosecond domain is measured with a scanning high-dynamic-range third-order cross-correlator (Sequoia, Amplitude Technologies)[18].
The Sequoia measurement results are shown in Fig.
Figure
The multiple pulses may have an impact on the laser–plasma interaction in two folds. The preceding intense pulses may produce a pinching magnetic field to collimate the successive laser-produced fast electrons[19,20]. Spectral enhancement of the laser-accelerated proton beam may be achieved with double pulses[21,22]. On the other hand, prepulse-free lasers are of prime importance for many topics of laser–solid interactions[9,10,23]. The temporal-shaped laser pulse with a sharp front is required to satisfy a near-solid-density short-scalelength plasma[24,25].
The measured laser temporal profiles with respect to

Fig. 2. Wigner distribution (left axis) and temporal profiles (right axis) of the laser pulse after reflection from the PM with equal to (a) , (b) , (c) , (d) , (e) , and (f) . The intensity is normalized to the peak value at . The shaded area in each plot is due to the shot-to-shot fluctuations.
Though the main peak pulses are similar in Fig.
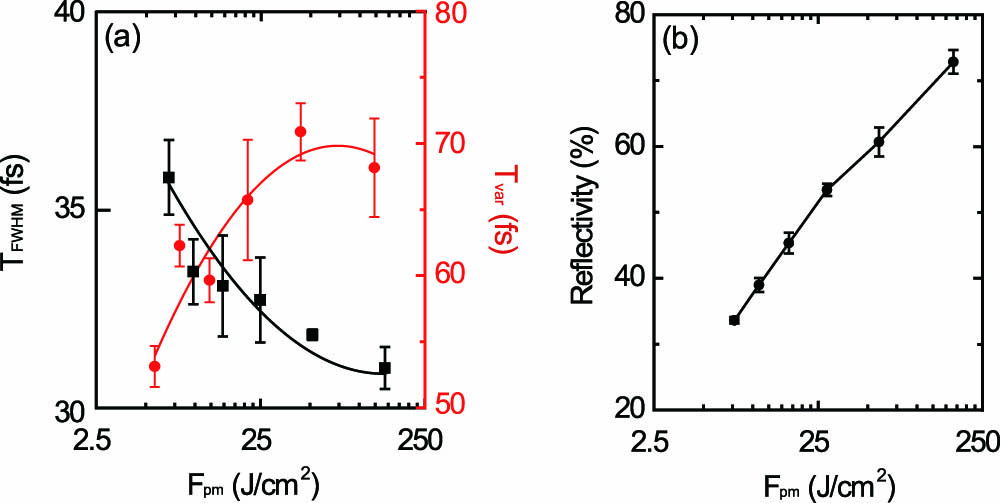
Fig. 3. (a) Pulse durations measured with FWHM ( ) and the second-order moment ( ) methods. The solid lines are drawn to guide eyes. (b) Integrated reflectivity of the PM-reflected laser pulse as a function of .
To understand the pulse shape change, the spectral intensity and phase of PM-reflected laser pulses from the retrieved FROG trace are compared in Figs.

Fig. 4. Retrieved spectral intensity (red line) and phase (black line) of the PM-reflected laser beam with the same laser fluence as in Fig. 2 . The shaded area in each plot is due to shot-to-shot fluctuations.
To further understand the spectral modulations presented in Fig.
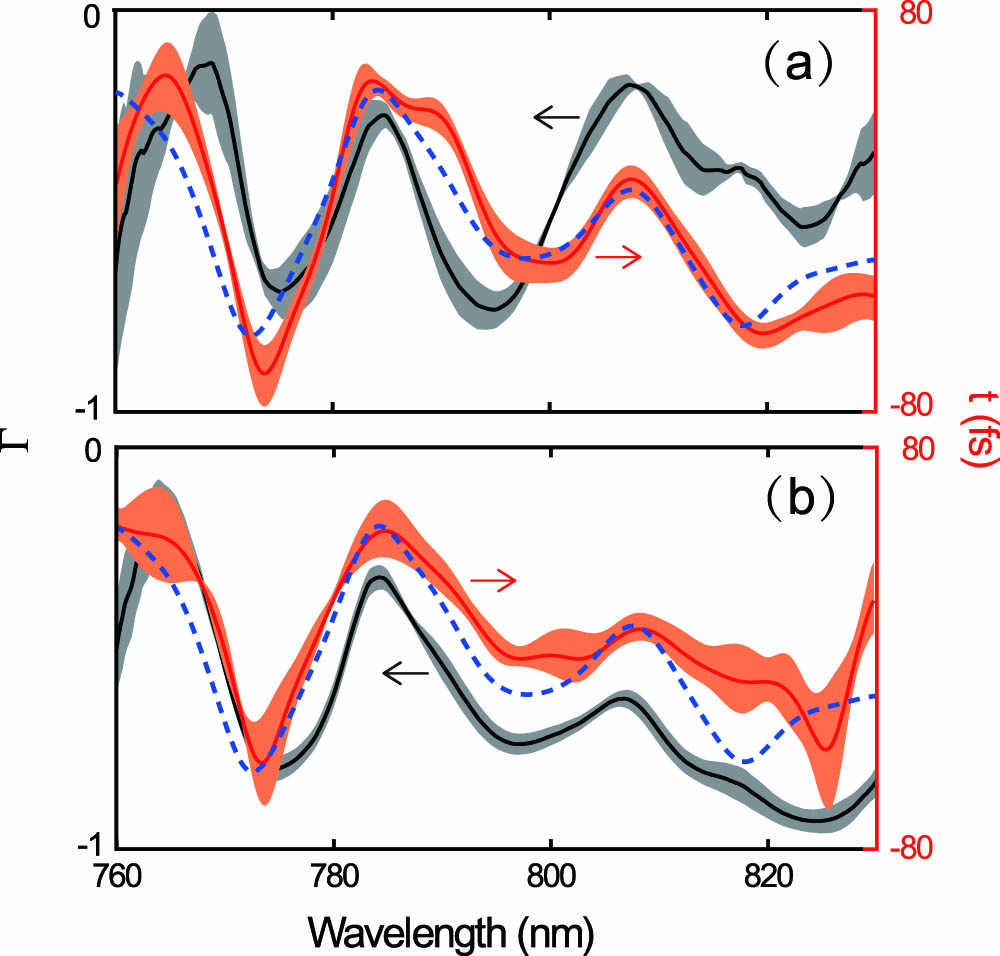
Fig. 5. Modulation depth (black line) and group delay (red line) for two laser fluence examples of (a) and (b) . The dashed lines are the reference group delays for the laser pulse without a PM. The shaded areas are the standard deviations due to shot-to-shot fluctuations.
In summary, we have measured the temporal profiles of laser pulses after reflection from a self-induced PM in the sub-picosecond temporal window. We find that the prepulses within a few hundred femtoseconds before the main peak could be suppressed by varying the fluence on the PM. The suppression is determined by the group delay of the main pulse. Experimental results suggest that special cares should be taken when non-ideally compressed pulses are used in experiments. Our findings will extend our knowledge on the proper utility of the PM technique for temporal contrast improvement and in applications of laser-driven particle acceleration and radiation source development.
[1]
[2]
[3]
[4]
[5]
[6]
[7]
[8]
[9]
[10]
[11]
[12]
[13]
[14]
[15]
[16]
[17]
[18]
[19]
[20]
[21]
[22]
[23]
[24]
[25]
[26]
[27]
[28]
Xulei Ge, Xiaohui Yuan, Yuan Fang, Wenqing Wei, Su Yang, Feng Liu, Min Chen, Li Zhao, Zhengming Sheng, Jie Zhang. Pulse shape of ultrashort intense laser reflected from a plasma mirror[J]. Chinese Optics Letters, 2018, 16(10): 103202.