Dark dimer mode excitation and strong coupling with a nanorod dipole
Download: 800次
1. INTRODUCTION
Surface plasmons, collective photon-electron coupled oscillations on the surface of metal nanostructures, offer an attractive method to manipulate light at subdiffractive scale [1]. Metallic nanoparticles support localized surface plasmon resonance (LSPR) with enhanced near field in their vicinity, showing remarkable potential in plasmon laser [2], biosensing [3], and quantum applications [4,5]. The LSPR interaction in coupled metal nanoparticles exhibits similar characteristics as the hybridization of electron wave functions in atomic orbitals; therefore, metal nanoparticles can be referred to as “plasmonic atoms” [6]. In recent years, mimicking classical atomic and molecular phenomena in plasmonic systems has been an important research topic to realize metallic nanostructures with tailored optical properties, for example, plasmonic analog of electromagnetically induced transparency (EIT) in a metasurface for the slow-light effect [7] and Fano resonance in an engineered plasmonic atom for directional light scattering [8] and high-sensitivity molecular sensing [9].
A plasmonic dimer is a pair of closely placed metal nanoparticles, which has been widely studied due to its geometric simplicity and resonance tunability. The bonding hybridization of dipolar resonance in each nanoparticle creates a “hot spot” where the electromagnetic field in the dimer junction is greatly enhanced [10,11]. The hot spot effect in plasmonic dimers has been exploited in boosting optical nonlinearity [12], the spontaneous emission rate of quantum emitters [13], and detection sensitivity towards single-molecule levels [14]. The antibonding interaction of dipolar resonances, on the other hand, results in a hybridized dimer mode with a vanishing dipole moment, which makes it difficult to interact with free-space radiation and thus appears as a dark mode [10,15]. The dark mode feature could also be found in high-order multipolar LSPR in single nanoparticles [16]. The suppressed radiation renders dark modes better able to store electromagnetic energy and is desired in plasmonic applications requiring lower loss [17], such as plasmon lasing [18,19] and plasmon-exciton coupling [20]. In addition, activating these dark modes by near-field excitation can also trigger Fano or EIT-like responses in coupled nanoparticles [21
Strong coupling in plasmonic systems is attracting growing research interest. The spectral splitting feature of strong coupling was observed in either metallic structures such as nanorod dimer lattices [26], plasmonic Fabry–Perot (F-P) cavity coupling with nanorods [27,28], and nanowire arrays [29], or dielectric-metallic hybrid systems such as nanorod-microfiber coupled cavities [30] and dielectric multilayer F-P cavities coupling to plasmonic disk dimers [31]. One approach to further facilitating strong coupling is lowering the dissipation rate, which could be achieved by employing nanostructures supporting dark modes without radiative decay.
In this paper, we numerically investigate dark dimer mode excitation and plasmonic strong coupling between dipole modes in a single gold (Au) nanorod, and antibonding dark mode in an end-to-end Au nanorod dimer, using the finite-difference time-domain method (Lumerical). We first studied excitation of bright and dark dimer modes using an electric dipole with different locations and dipole moment orientation. Next, utilizing the narrow-linewidth dark-mode resonance in a nanorod dimer, we show that plane-wave excited dipole resonance in a nanorod can be strongly coupled to the dark mode in a nanorod dimer through near-field interaction with the energy exchange rate surpassing the plasmonic decay; the dependence of spectral splitting on coupling distance is investigated and examined by the strong coupling criterion. Finally, we demonstrate the anticrossing behavior of the dipole nanorod mode and dark dimer mode as well as the Rabi-like oscillation of energy exchange between the strongly coupled modes.
2. RESULTS AND DISCUSSIONS
Au nanorods synthesized by low-cost bottom-up methods have the advantages of single crystalline and better morphology uniformity, which are highly desired building blocks for visible to near-infrared nanoplasmonic applications [32]. A single nanorod supports longitudinal plasmonic dipole modes, as depicted in Fig.
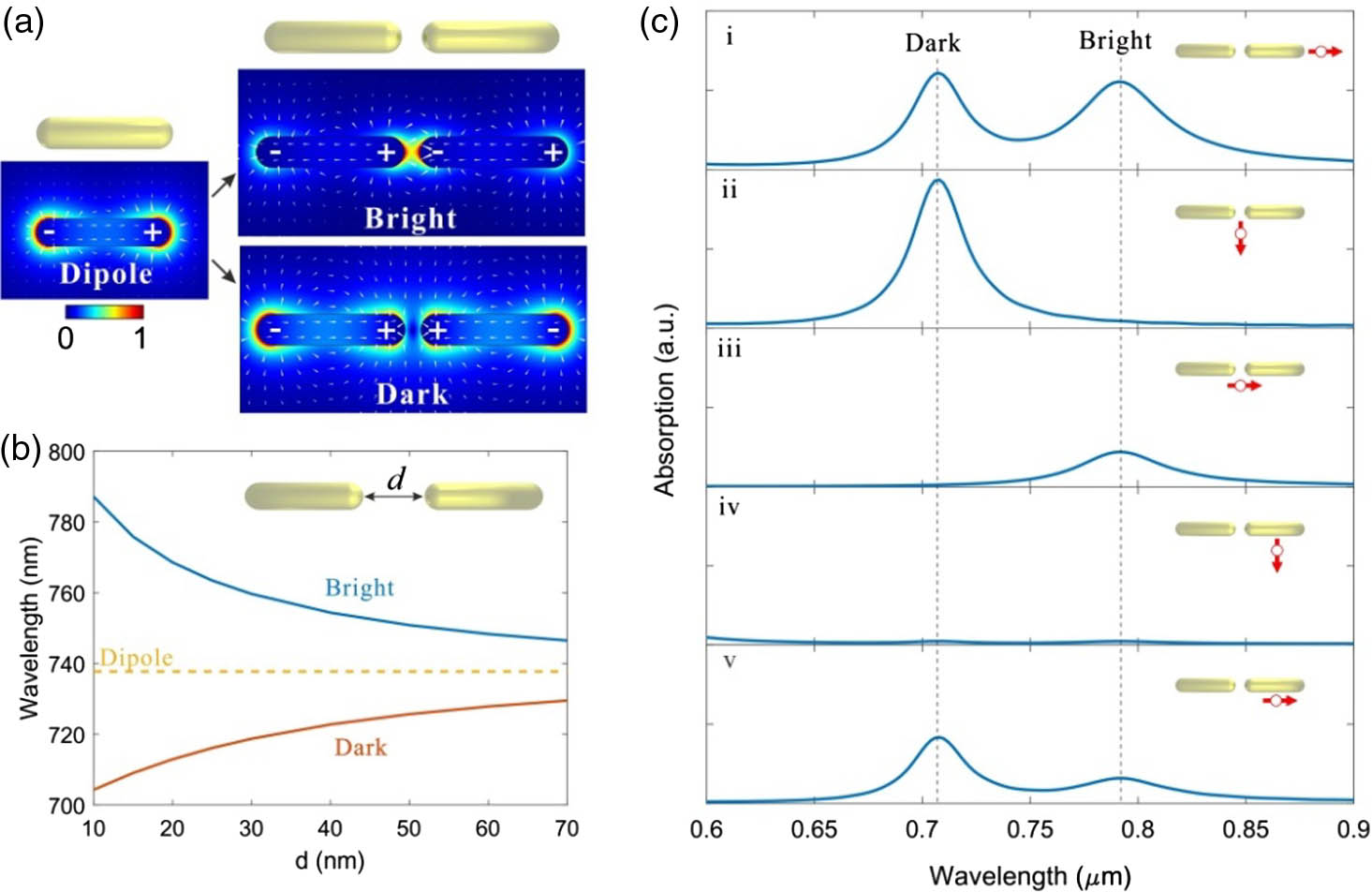
Fig. 1. Au nanorod dimer excitation with an electric dipole. (a) Mode profile and surface charge distribution of a longitudinal dipole mode in a single Au nanorod and hybridized bright and dark modes in a nanorod dimer; (b) resonant wavelengths of bright and dark modes as a function of dimer spacing . The yellow dashed line denotes the resonant wavelength of a dipole mode in a single rod. The length and diameter of each nanorod are 95 and 20 nm, respectively. (c) Absorption spectra of the Au nanorod dimer under different electric dipole excitation conditions. The dimer spacing is 10 nm. The electric dipole is located 20 nm away from the Au nanorod dimer. The red arrow indicates the electric dipole moment orientation and position relative to the nanorod dimer. The bright and dark modes are located at the wavelengths of 791.0 and 704.3 nm, respectively.
Figure
The above-mentioned results can be explained by the coupling factor
For real cases, we replace the electric dipole with an Au nanorod, forming the plasmonic strong coupling system studied in this paper, as shown in Fig.

Fig. 2. (a) Schematic of plasmonic strong coupling in an Au nanorod structure. A single nanorod is placed along the middle line of the nanorod dimer with a separation of , as denoted in the inset. A normally incident plane wave with a wave vector is linearly polarized along the axis of a single nanorod. (b) Absorption spectra of the dipole mode in a single nanorod (blue) and dark mode in a nanorod dimer (red). The rod length and spacing of the dimer are 95 and 30 nm, respectively. The length of the single nanorod is 90.4 nm. The diameter of all nanorods is 20 nm.
Figure
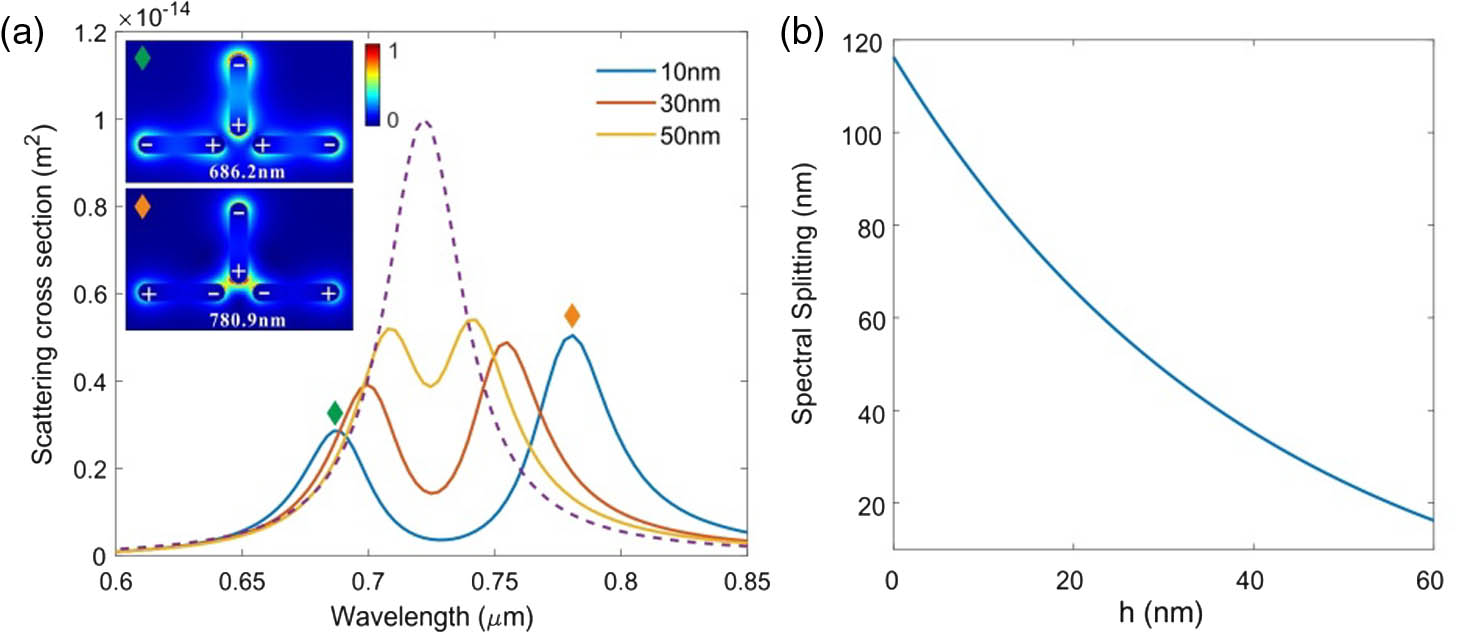
Fig. 3. (a) Scattering spectral splitting of the coupled nanorods with different coupling distance. The dashed curve shows the scattering of an individual dipolar nanorod. Electric field profiles of the coupled nanorods with a coupling distance at 686.2 and 780.9 nm. Plus and minus signs denote the surface charge distribution. (b) The dependence of spectral splitting on the coupling distance .
Anticrossing behavior is another characteristic signature for strong coupling. Figure
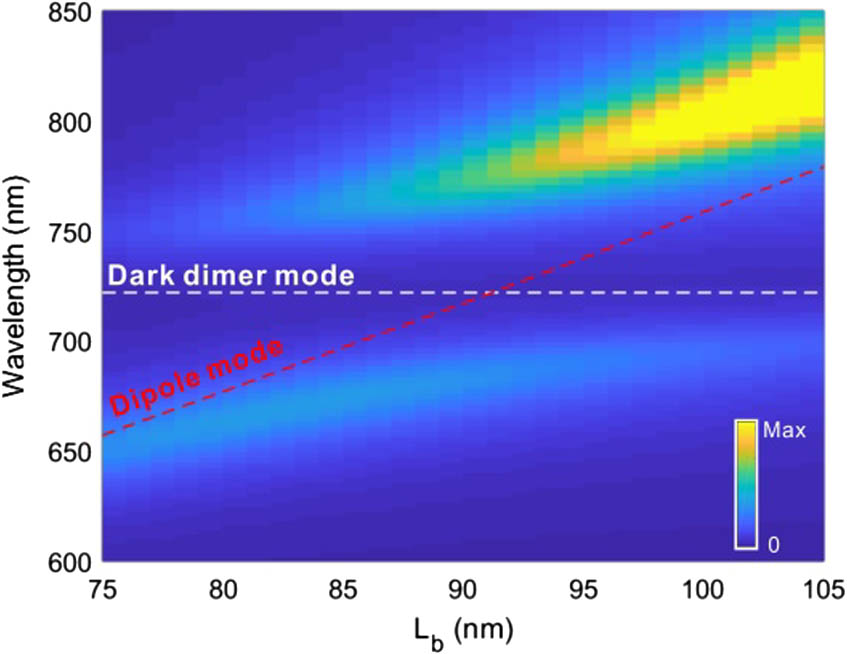
Fig. 4. Scattering spectra of the coupled nanorods with varying dipole resonator length . The white and red dashed lines correspond to an unperturbed dark dimer mode and the dipole mode. The saturated scattering intensity on the upper-right corner is provided intentionally to get better contrast for the lower scattering branch due to a much larger scattering of the dipolar nanorod with longer .
To gain a deeper physical insight into the strong coupling process, we plot temporal electromagnetic energy (represented by the electric field) exchange between the dipole mode and the dark dimer mode in Fig.
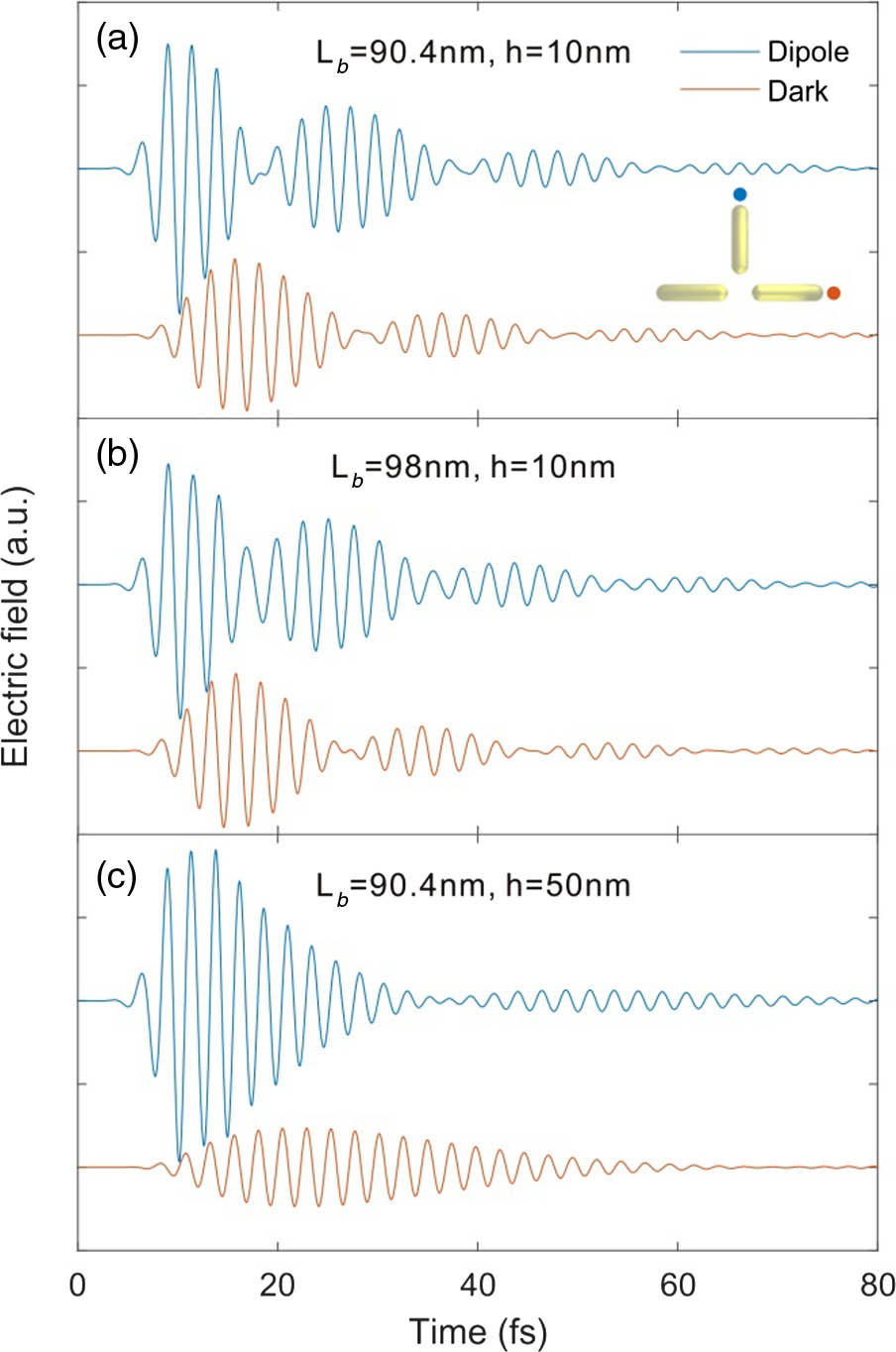
Fig. 5. Coherent energy exchange between the dipole mode and the dark dimer mode. (a) Complete energy exchange within the strong coupling regime, (b) partial energy exchange with a detuned frequency between the dipole and the dark dimer modes, (c) energy exchange with a lower exchange rate in the weakly coupled dipole and dark dimer modes. Time-dependent electric field amplitudes near the dipolar nanorod and the dark dimer are measured at the corresponding colored spots denoted in the inset of (a), which are 1 nm away from the rod end. The parameters of coupled nanorods are the same as in Fig. 3 , except and .
For the potential application of the proposal, first, we think it can be used as a highly compact approach to exciting and investigating dark modes in individual nanorod dimers with strong coupling. Second, as the spectral response is highly sensitive to material and environmental parameters [see, for example, Fig.
3. CONCLUSION
In summary, we studied plasmonic dark dimer mode excitation and its strong coupling with a nanorod dipole in Au nanorods. Selective excitation of bright and dark modes in a nanorod dimer can be achieved by a properly arranged electric dipole, which can be qualitatively determined by a coupling factor
Article Outline
Yixiao Gao, Ning Zhou, Zhangxing Shi, Xin Guo, Limin Tong. Dark dimer mode excitation and strong coupling with a nanorod dipole[J]. Photonics Research, 2018, 6(9): 09000887.