Noise-sidebands-free and ultra-low-RIN 1.5 μm single-frequency fiber laser towards coherent optical detection
1. INTRODUCTION
In recent years, single-frequency fiber lasers have been extensively studied because of some advantages such as high beam quality, all-fiber configuration, and narrow linewidth, which enable them to be extensively applied in various fields, including nonlinear frequency conversion, high-precision spectroscopy, coherent beam combing, and laser detection [1–
In coherent light detection based on single-frequency fiber lasers, it can be found that two noise peaks exist on both sides of the detected signal [10]. The noise peaks are called noise sidebands. The presence of noise sidebands limits the measurement results and the minimum detectable signals in coherent light detection [11,12]. For single-frequency fiber lasers, previous theoretical research has briefly reported that RIN and frequency noise at the relaxation oscillation frequency
With regard to RIN, even if a well-designed continuous wave (CW) free-running laser may be sufficiently stable for most applications, amplitude stabilization is still required to achieve the high performance required in a coherent detection system, which is conducive to distinguish weak signal from a complex radio-frequency spectrum [14,15]. Regarding RIN suppression, a common technology is the optoelectronic feedback method, while the suppressed amplitude and bandwidth are restricted by the natural inferior property of the optoelectronic feedback loop [16]. Another technique to reduce RIN is based on a mode-cleaner with feedback loop [17]. Nevertheless, the mode-cleaner, suffering from environmental sensitivity and voluminous architecture [18], increases the operation difficulty and causes mismatch between the spatial light structure and the all-fiber configuration of the single-frequency fiber laser.
Recently, we have presented broad-bandwidth near-shot-noise-limited intensity noise suppression of a single-frequency fiber laser based on a semiconductor optical amplifier with optoelectronic feedback [19]. In this paper, we introduce a noise-sidebands-free and ultra-low-RIN 1.5 μm linearly polarized single-frequency fiber laser. With the comprehensive effect of a self-injection locking (SIL) system and a booster optical amplifier (BOA), not only are the noise sidebands with relative amplitudes of 20 dB completely suppressed, but the RIN is also reduced to below
2. EXPERIMENTAL SETUP
The experimental setup of the noise-sidebands-free and ultra-low-RIN 1.5 μm single-frequency fiber laser based on BOA and SIL is displayed in Fig.
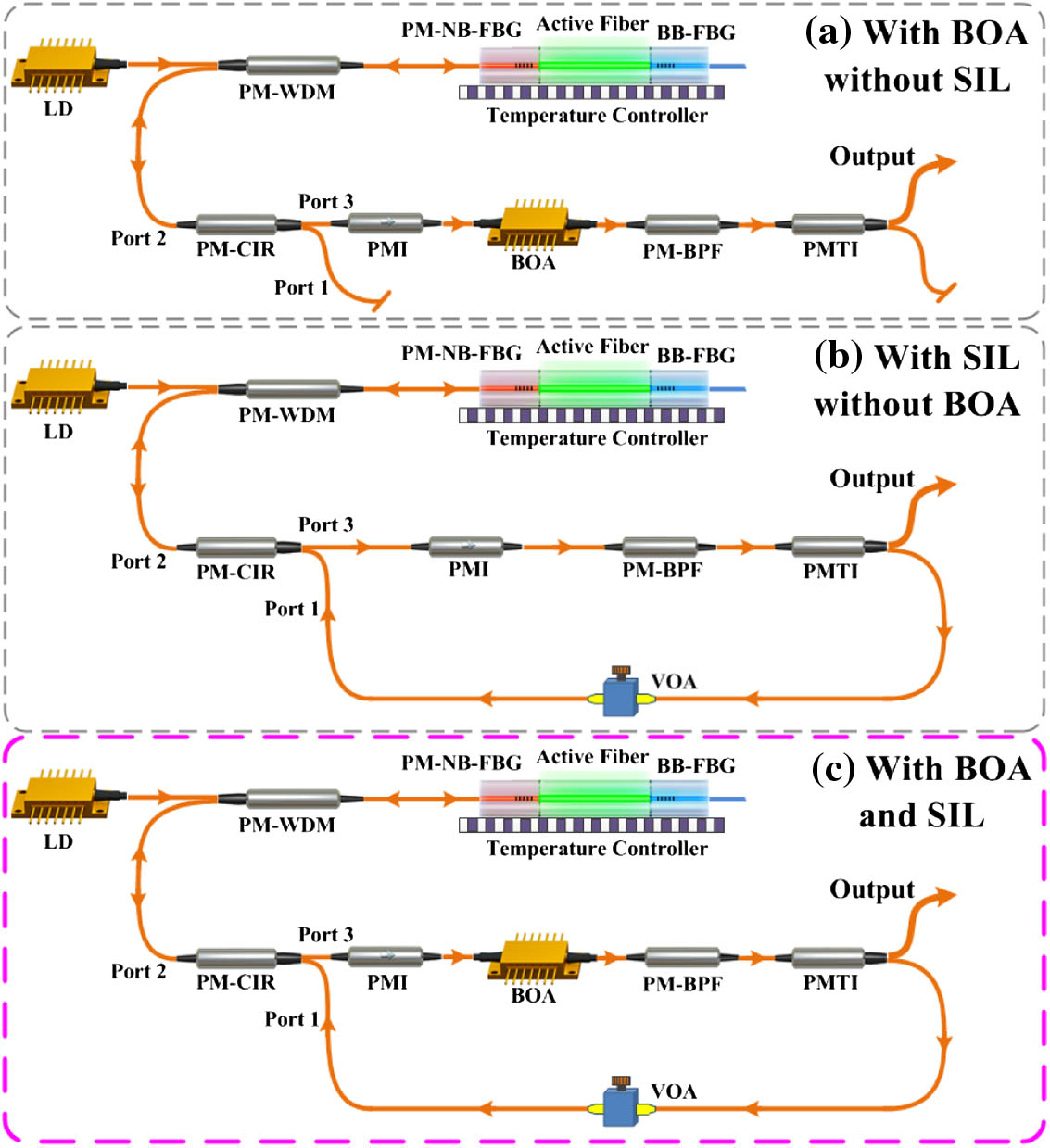
Fig. 1. Experimental setup in different structures (a) with BOA without SIL, (b) with SIL without BOA, and (c) with BOA and SIL. FBG, fiber Bragg grating; PM-NB-FBG, PM narrow-band FBG; BB-FBG, broadband FBG; LD, laser diode; PM-WDM, PM wavelength division multiplexer; PM-CIR, PM circulator; PMI, PM isolator; PM-BPF, PM bandpass filter; PMTI, PM tap isolator; VOA, variable optical attenuator.
The laser cavity is temperature-controlled through a cooling system with a resolution of 0.05°C, and it is pumped via a 980 nm single-mode laser diode with a PM wavelength division multiplexer. The laser from the PM wavelength division multiplexer is then launched into port 2 of a PM circulator. Port 3 of the PM circulator and the input port of a BOA are connected by a PM isolator, which is used to guarantee unidirectional transmission of the laser. The BOA is a high saturation output power, high bandwidth, low-ripples, and PM optical amplifier, with a central wavelength of 1550 nm, a 3 dB gain bandwidth of 85 nm, a saturation output power of 15 dBm, and a small signal gain of 27 dB. It incorporates a highly efficient InP/InGaAsP quantum well layer structure and a reliable ridge waveguide design. A designed PM bandpass filter (3 dB bandwidth: 1 nm) is added to filter out the amplified spontaneous emission brought in by the BOA. Then a 10/90 PM tap isolator, combining a PM tap coupler and a polarization-sensitive isolator into a single compact package, is employed to split 10% of the laser power through a variable optical attenuator launching into the port 1 of the PM circulator, which renewedly enters the laser cavity to realize the SIL. The 90% port of the PM tap isolator is the final laser output. All the experimental optical paths are well fixed on the optical table with special optical glue to reduce the unnecessary acoustic vibrations from the external environment.
During the experiment, the driving current of the laser diode is 250 mA, corresponding to the 14 mW laser output power. The laser power feeding into the BOA is 12 mW, while the driving current of the BOA is set to 200 mA. The power injected to the PM circulator is adjusted to 1 mW by the variable optical attenuator. Ultimately, the output power from the 90% port of the PM tap isolator is 13 mW.
The measurement scheme of coherent light detection is based on a Mach–Zehnder interferometer. A 30/70 optical coupler splits the output laser into two beams. The 30% beam is frequency shifted by an acousto-optic modulator, while the 70% beam is time delayed by a delay fiber. Then a 50/50 optical coupler combines two laser beams launching into a high-speed photodetector to acquire the detected signal.
3. RESULTS AND DISCUSSION
In the experiment, various technologies, i.e., BOA only, SIL only, and BOA combined with SIL, are investigated with respect to the RIN suppression and noise sidebands elimination. The resultant RINs in different frequency ranges are compared with the quantum noise limit as shown in Fig.

Fig. 2. RIN spectra of this single-frequency fiber laser with different conditions. The quantum noise limit of is also shown for comparison. (a) 0 to 5 MHz. (b) 0 to 50 MHz.
Significantly, with the comprehensive effect of BOA and SIL, the RIN peak is remarkably reduced by more than 64 dB from
Utilizing the single-frequency fiber laser with different statuses, the signals of coherent light detection are measured seriatim as shown in Fig.

Fig. 3. Measured signals of coherent light detection of this single-frequency fiber laser in different statuses.
Interestingly, with the SIL scheme, a maximum RANS suppression of more than 20 dB is realized, and two noise sidebands are absolutely reduced. The reasons for this action in the SIL laser are twofold. First, the resultant increasing photon lifetime reduces the RIN level [25]. The photon lifetime, which is the time needed for the light field to travel back and forth across the whole cavity, is extended by the introduction of the SIL loop [21]. Second, the SIL system drives a strong lasing frequency pulling, which causes the threshold gain difference of each mode [26]. This effect can promote the laser energy to concentrate on the main laser signal, and reduce the amplitude of the noise sidebands. Benefitting from the above two properties, only the main signal of the coherent detection exists.
Similarly, the coherent detected signal of the single-frequency fiber laser with SIL and BOA is also measured. The signal curve is almost coincident with that in the SIL scheme without BOA, and these noise sidebands also disappear. This indicates that the admirable performance of the coherent signal is not deteriorated by the insertion of the BOA in the SIL laser, thereby unveiling the unique advantage of the SIL technique in controlling the noise sidebands.
An optical spectrum with high optical SNR contributes to reducing the noise floor of the coherent detected signal. So the optical spectra are recorded at different output ports with a scanning span of 50 nm and a resolution bandwidth of 0.02 nm. To avoid a deterioration of optical SNR caused by the BOA-induced amplified spontaneous emission, a PM bandpass filter is employed, and the filtered spectrum is exhibited in Fig.
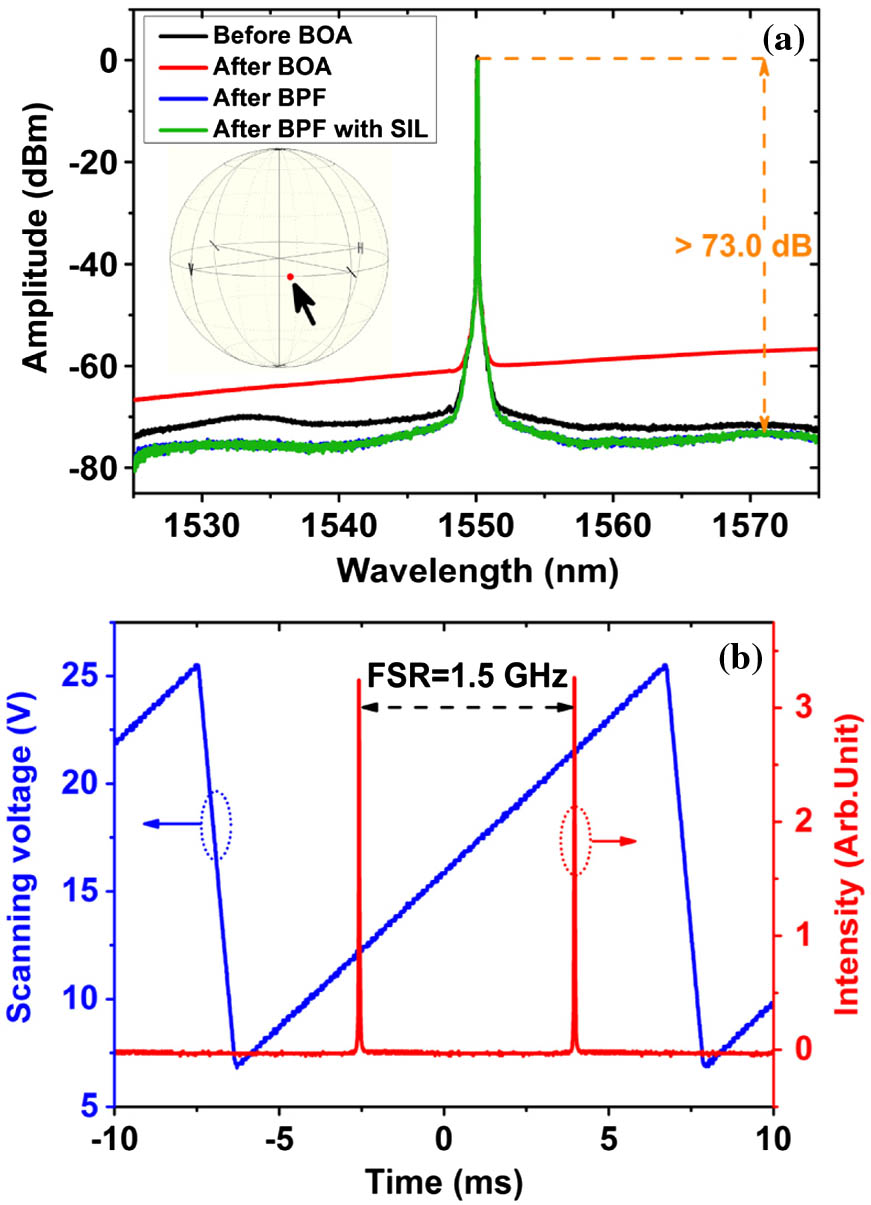
Fig. 4. (a) Optical spectra of this fiber laser at different output ports. The inset shows the degree of polarization of the final laser output (red dot) represented by a Poincaré sphere. (b) Measured single-longitudinal-mode characteristic of the final laser output.
A single-longitudinal-mode working of the laser source is a basic requirement of coherent optical detection. Thus, the single-longitudinal-mode characteristic of this fiber laser after BOA with SIL is tested by a scanning Fabry–Perot interferometer with a resolution of 7.5 MHz and a free spectral range of 1.5 GHz. From Fig.
The linewidth of the laser source is also momentous in coherent optical detection, especially for long-distance applications. The linewidths are evaluated by the self-heterodyne method involving a 48.8 km fiber delayed Mach–Zehnder interferometer and a 40 MHz fiber coupled acoustic optical modulator. Figure
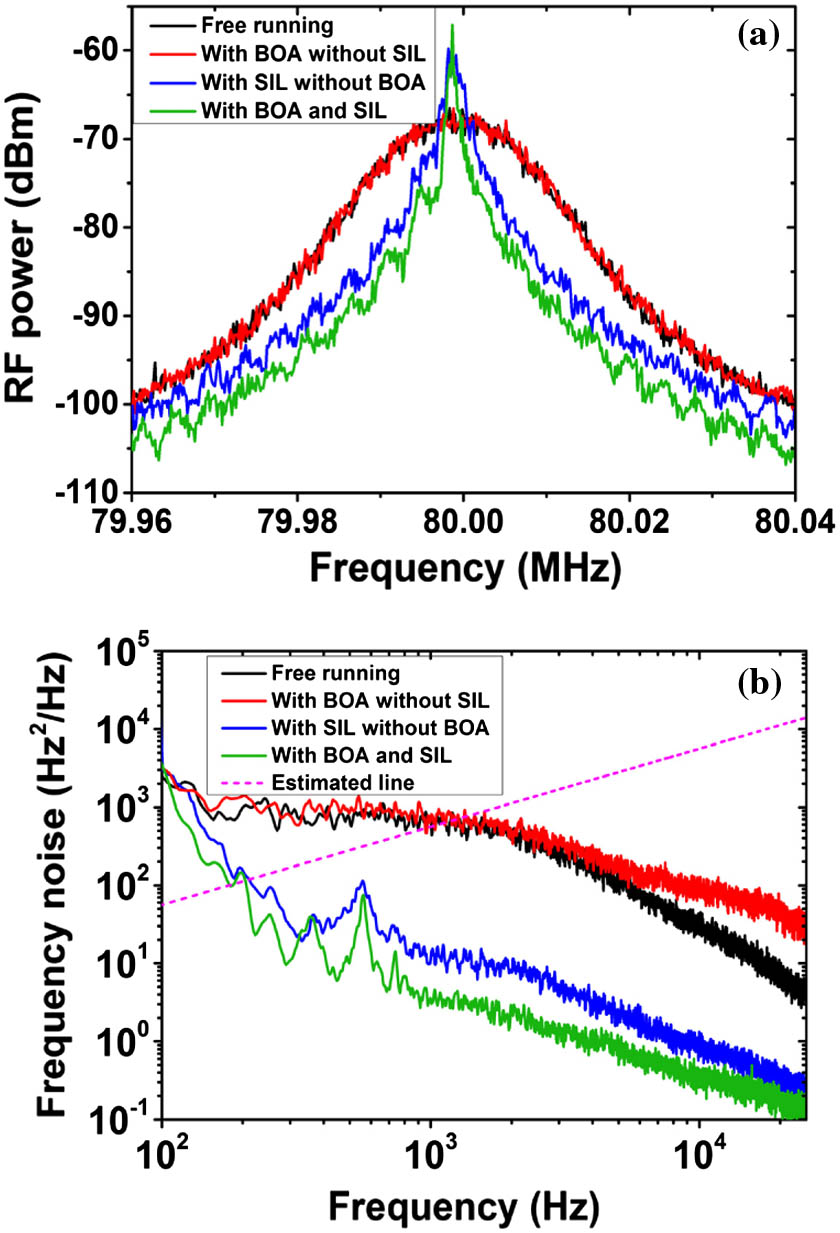
Fig. 5. (a) Measured self-heterodyne spectra of this fiber laser with different conditions. (b) Measured frequency noise spectra and estimation line for evaluation of linewidth.
To overcome the limitation of the self-heterodyne method and obtain a more accurate laser linewidth, the frequency noise spectra are measured via a fiber Michelson interferometer with 100 m optical path difference and an optical phase demodulator [27]. Figure
4. CONCLUSIONS
We present a noise-sidebands-free and ultra-low-RIN 1.5 μm single-frequency fiber laser for the first time to our best knowledge. By means of the SIL technique combined with a BOA, the RANS is reduced by more than 20 dB and the noise sidebands are completely suppressed. A maximum reducing amplitude over 64 dB of the RIN is obtained, and the RIN is dramatically suppressed to be below
Qilai Zhao, Zhitao Zhang, Bo Wu, Tianyi Tan, Changsheng Yang, Jiulin Gan, Huihui Cheng, Zhouming Feng, Mingying Peng, Zhongmin Yang, Shanhui Xu. Noise-sidebands-free and ultra-low-RIN 1.5 μm single-frequency fiber laser towards coherent optical detection[J]. Photonics Research, 2018, 6(4): 04000326.