Ion source perturbation and control in intense laser plasma interaction
1 I. INTRODUCTION
The acceleration of high-energy ion beams (up to several tens of mega-electronvolts per nucleon) following the interaction of ultra-short (t < 1 ps) and intense (Iλ2 > 1018 W cm−2) laser pulses with solid targets has been an active research area in the last few years. Mechanisms leading to forward-accelerated high-quality ion beams, operating at the currently accessible intensities in laser–matter interactions, are mainly associated with the large electric fields created by laser-accelerated electrons at the target interface. The emitted ion pulses contain many particles (up to 1013) with energies in excess of several mega-electronvolts,
The above outstanding characteristics of laser-accelerated ion beams have triggered discussions about their application as an ion source injected into conventional particle accelerators.
The unique properties of protons from high-intensity laser–matter interactions, particularly in terms of spatial quality and temporal duration, have opened up the totally new applications of proton probing and proton radiography.
Recent experiments
2 II. EXPERIMENTAL DETAILS
The experiments were carried out using a 10-TW Ti:sapphire laser system at the Raja Ramanna Centre for Advanced Technology, Indore, which delivered 50-fs (FWHM) 420-mJ pulses at a 10-Hz repetition rate at a central wavelength of 800 nm. The p-polarized laser beam was focused to a focal spot 10 μm in diameter (FWHM) by a gold-coated f/7.5 off-axis parabolic mirror to give a peak intensity of the order of ∼2 × 1018 W/cm2. A schematic diagram of the experimental setup is shown in
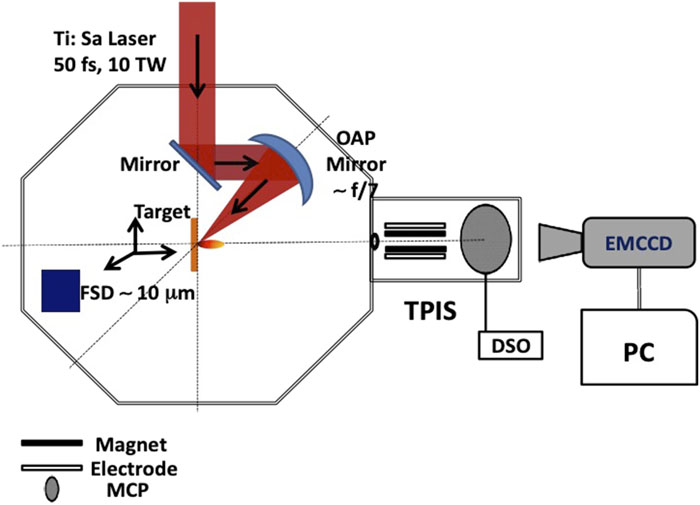
Fig. 1. Experimental setup. The laser (λ = 800 nm) was focused by an off-axis parabola (f/7.5) at an angle of 45° to the target. It produced up to 400 mJ in 45 fs with peak intensities ∼2 × 1018 W cm−2.
The angle of incidence of the laser on the target was set to 45° to allow efficient absorption of the laser pulse.
3 III. RESULTS AND DISCUSSION
Now we discuss the possibility of controlling the pointing of accelerated ion beams by adding a pre-pulse to the main pulse of the laser. The wiggling of the proton traces was studied in detail by varying the pre-pulse conditions, for Perspex [poly(methylmethacrylate) or PMMA], Mylar (CHn), and deuterated polyethylene (CD2) targets, which are observed by the TPIS detector.
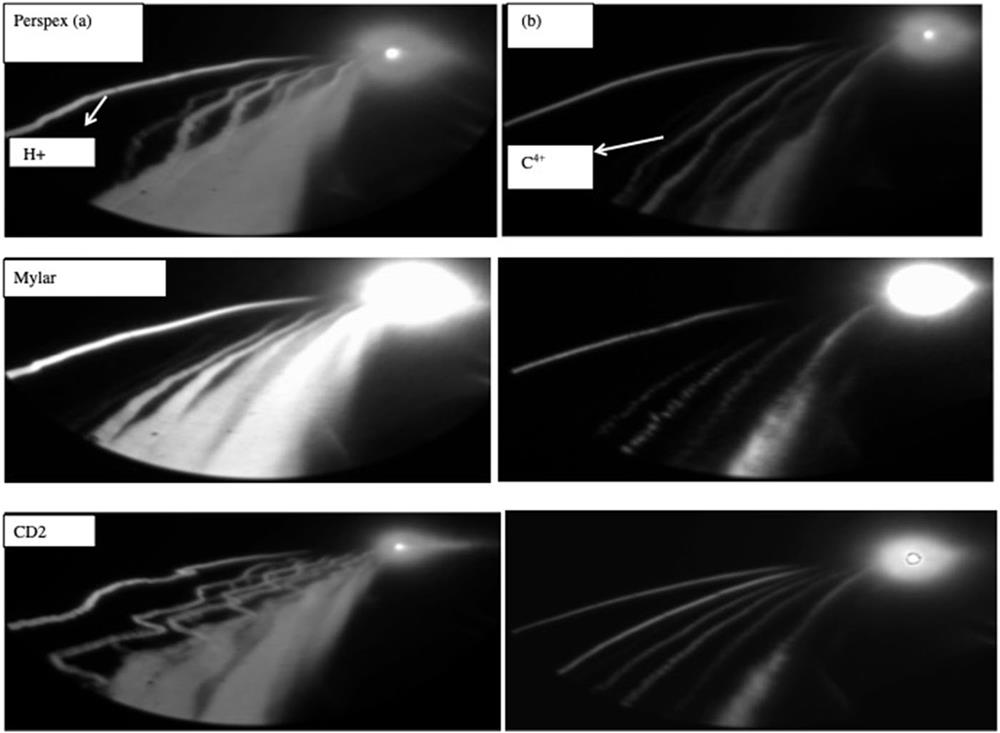
Fig. 2. Proton beam traces from the front surfaces of Perspex (top), Mylar (center), and CD2 (bottom) targets. The images show the wiggling of the beam with the target at the best focus, (a) without a pre-pulse and (b) with a pre-pulse.
When the ASE pre-pulse was extended by changing the switch-out time of the pulse selector in the laser, the wiggling features in the spectral trace were considerably reduced, with no reduction in the maximum ion energy [
The wiggling of the traces in our case could be attributed to the presence of multiple sources, which create perturbations in the accelerating sheath (in the absence of a pre-pulse). The pre-pulse wipes out the source multiplicity occurring at ps time scales. Thus, one can control the ion source perturbations in a laser plasma and mitigate the effects by varying the extent of the laser pulse. In other words, by using an extended pre-pulse, one can eliminate wiggling from the proton spectral trace. However, the wiggling of the heavier ion beams in our experiment could not be controlled very effectively using this technique, due to their slow response to the sheath field. Note that we used the same experimental and diagnostic conditions for experiments both with and without the laser pre-pulse, and our results confirm that there were no experimental artifacts due to the detector.
As reported in the literature,

Fig. 3. (a) Laser pre-pulse contrast at various Pockels cell settings. (b) A typical proton (H+) energy spectra recorded for a pre-pulse on a Perspex target showing the 1-MeV cutoff.
The laser intensity and energy determine the pulse of the hot electrons circulating through the target and the building up of the acceleration sheath at the front of the target. As described previously, ions are accelerated by the electron sheath at the front. The spatial and angular characteristics are determined by the electron density distribution within the sheath. At higher energies, the proton beam has an angular distribution with a sharp boundary. The electron sheath follows a generic bell-shaped spatial distribution. The high-energy protons are accelerated from the tip of the sheath with a very small divergence angle. The acceleration of low-energy protons occurs from larger regions, including the wings of the sheath, and hence, a large divergence is observed. The virtual source position in front of the target changes for different proton energies, which implies that protons with different energies are emitted with an accelerating sheath field with a different curvature. In Ref.
4 IV. SIMULATIONS
Two-dimensional particle-in-cell (PIC) simulations were performed using the PIC code Osiris.
The numerical parameters were as follows. In the hard-edge simulations, the simulation box measures 6672 × 1248 cells and is 32 × 32 µm2. In the gradient simulations, the simulation box measures 10 000 × 1872 cells and is 48 × 48 µm2. In all simulations, the number of particles per cell was 20 for electrons, 8 for protons, and 12 for carbon. The time step was 15.4 as, to satisfy Courant’s condition. In all simulations, the laser pulse had a wavelength of 0.8 µm, an intensity of 2 × 1018 W/cm2, a duration of 30 fs, and a focal spot diameter of 10 µm. The laser pulse was focused onto the center of the front surface of the foil. The direction of propagation was 45° with respect to the target normal. To simplify the simulations, it was decided to make the laser propagate in the x1 direction by rotating the target by 45°. In all simulations, the target was 10 µm thick and 30 µm wide. The peak target electron density was 10 times the critical density for the laser beam, i.e., 1.1 × 1022 cm−3. The target ion composition was H+ and C4+ in a ratio of 2:1. The target ion density was such that the entire target was charge neutral.
For the hard-edge target, the density profiles in the target normal and transverse directions were rectangular. The density profile for the gradient target was much like that of the hard-edge target, but with a density gradient at the front surface (facing the laser pulse). Radiation-hydrodynamic simulations of the pre-pulse interaction were performed using good approximations to the actual experimental parameters. This resulted in significant hydrodynamic expansion. The resulting density profile was fitted so that it could be mapped into a PIC code. The gradient extended for about 15 µm in the target normal direction, and the electron density rose linearly from 0 cm−3 to 1.1 × 1022 cm−3, with matching ion densities. As discussed above, this density gradient mimics the plasma expansion due to preheating by the 2-ns pre-pulse.
The simulation results are shown in
![Two-dimensional PIC simulation results. (p2,p1) plots of proton ions at various times: [(a) and (b)] without a pre-pulse and [(c) and (d)] with a pre-pulse.](/richHtml/mre/2020/5/4/045402/img_4.jpg)
Fig. 4. Two-dimensional PIC simulation results. (p 2,p 1) plots of proton ions at various times: [(a) and (b)] without a pre-pulse and [(c) and (d)] with a pre-pulse.
The classic Thomson parabola can be reproduced only if all protons enter the TPIS at the same angle and transverse position. In particular, the last few phase plots from the hard-edge target show a proton bunch being emitted with a strong correlation between proton speed and transverse position along the target. The difference in results between the two targets can be explained as follows. For the hard-edge target, the laser pulse cannot penetrate very far into the target. The pulse mostly heats the target electrons, which then expand and form a sheath. There is little interaction between the accelerated protons and the laser pulse.
For the gradient target, however, the laser pulse can penetrate the low-density plasma in front of the foil. A large fraction of the accelerated protons originate from the low-density gradient in front of the target, rather than from the high-density interior part of the target. It is hypothesized that this interaction between the main laser pulse and the protons accelerated by the pre-pulse form a smooth sheath, and hence, there is an unperturbed source accelerated by the Coulomb force.
To understand the pointing of the laser-accelerated proton beams, it is very important to have a detailed understanding of the temporal evolution of the acceleration process. Our study can be further improved by using PIC simulations to understand the variation in the angular beam emittance and perturbation for the various targets described in this paper.
5 V. CONCLUSION
In conclusion, we studied the ion emission from the front surface of solid targets interacting with terawatt laser pulses. Our study indicates that proton emission from the target front surface is not uniform due to the perturbation in the source arising from intense laser plasma interactions. The wiggling of the beams was further characterized by using thick plastic targets. We found that the maximum source perturbation of protons occurred for plastic targets. We investigated a technique to control this by manipulating the laser parameters, specifically, by adding a proper pre-pulse to the main laser pulse. Two-dimensional PIC simulations were performed for the CH targets with and without pre-pulses. The simulations agree well with the experimental results. The introduction of a long pre-pulse smears out any perturbations of the ion source, resulting in a well-defined steering of the ion beam. This study could improve our understanding of ion acceleration and aid in using these directed proton beams for fast ignition fusion by depositing the maximum amount of energy in the core to produce viable fusion energy.
Article Outline
B. Ramakrishna, S. Krishnamurthy, M. Tayyab, S. Bagchi, K. Makur, Raoul Trines, Robbie Scott, Alex Robinson, J. A. Chakera. Ion source perturbation and control in intense laser plasma interaction[J]. Matter and Radiation at Extremes, 2020, 5(4): 045402.