氦原子在超短中红外脉冲和极紫外光场作用下的双电离
下载: 836次
1 Introduction
Thanks to the great advances of ultrashort laser technologies, ionization of atoms and molecules in strong laser fields has been extensively studied in past decades. On the basis of the understanding of single ionization, which can be grouped into multiphoton ionization[1-2] or tunneling ionization[3-5] according to the Keldysh parameter[6], double ionization has also attracted attention during the past years. As the simplest two-electron system, the helium atom has worked as a prototypical system for understanding double ionization, as well as the multi-electron atom Mg[7-8]. When a helium atom is exposed to extreme ultraviolet (EUV) pulses, one electron may absorb high energetic photons and escape from the parent ion, meanwhile the bound electron is shook off[9]. The atom may simultaneously absorb two photons if one is not enough, and the photon energies are shared by the two freed electrons[10]. For a helium atom in intense long-wavelength laser fields, the concept of field ionization is more vivid. In this case, the two electrons may eject in two independent single ionization processes[11], which is termed as “sequential double ionization” (SDI). Alternatively, the two electrons may be also stripped off via nonsequential double ionization (NSDI), i.e., one electron tunnels out of the laser-distorted Coulomb potential, and later rescatters with its parent ion, ultimately resulting in double ionization. Depending on the rescattering energy, the second electron may be kicked off directly, identified as recollision-impact ionization (RII), or be pumped to some excited states, which will be tunneling ionized in the remaining laser field, as termed as recollision excitation with subsequent ionization (RESI)[12-13]. NSDI can be described reasonably well by the recollision model[14]. The SDI and NSDI dominate in different laser intensity ranges. Sometimes, the ionized electron that does not gain sufficient drift momentum in this process would be recaptured by the Coulomb attracting potential and eventually be trapped into the high-lying Rydberg states, which we called as“frustrated tunneling ionization (FTI)”[15-16].
In the two-electron joint momentum distribution, RII contributes to the momentum distribution in the first and third quadrants[17-19]. As intensities are below or close to the recollision threshold, anticorrelation between the electrons has been observed[20-22]. Electron anticorrelation was basically explained within the mechanisms of recollision-induced-excitation tunneling and multiple-recollision[23-25]. The recollision-induced-excitation tunneling mechanism always prefers to cause back-to-back emission[24]. In the multiple-recollision case, the ionization events uniformly distribute in the four quadrants of the electron-electron momentum distribution diagram[26], and the sum-energy spectrum of two electrons can be fitted with the Maxwellian distribution[27]. If several excited states are mediated in the double ionization process, the interference from different excitation channels will break the fourfold symmetry of the RESI distributions[28].
In spite of many intriguing phenomena discovered in the double ionization, some questions still remain open. For example, may NSDI and SDI coexist and interfere with each other in some laser conditions? Are there some new phenomena for the double ionization if the driving laser pulse is extremely short?
The theoretical study of double ionization of helium or other more complex systems relies on the numerical simulation of the time-dependent Schrödinger equation, or the strong field approximation, or even time-dependent perturbation theory if only few photons are involved. The fully dimensional time-dependent Schrödinger equation (TDSE) simulation for helium in infrared or MIR laser fields is still overloaded for supercomputers. However, the reduced dimensional TDSE can capture the main dynamics and thus is still used in many research[29-33]. In this paper, we numerically simulate the TDSE by confining both electrons moving along the laser polarization axis and study the correlated and anticorrelated electron joint momentum distributions. Physically, one electron absorbs a high-energetic EUV photon and gets released, and the MIR field may drive it back to He+, resulting in the NSDI. Alternatively, after the single ionization of He, the new produced He+ may be further tunneling ionized directly by the MIR field, resulting in the SDI. The interference of NSDI and SDI is expected and demonstrated in the correlated joint electron momentum distribution. Surprisingly, the two electrons freed via rescattering may also propagate oppositely.
2 Numerical models
We have performed
as a sum of the field-free Hamiltonian
with
where
where
The laser-electron interaction is expressed in length gauge:
where
with a Gaussian envelope
In simulations, we set the spatial grids Δ
correlated: |
anticorrelated: |
In simulations, we propagated the wave function for an extra 200 a.u. after the laser field vanished in order to wait for all double ionized components to enter the partitioned areas. At the end of propagation, electron-electron momenta and energy spectra of double ionization were obtained by Fourier transforming the wave function distributed in partitioned areas. The initial state
3 Simulation results
3.1 Correlated double ionization
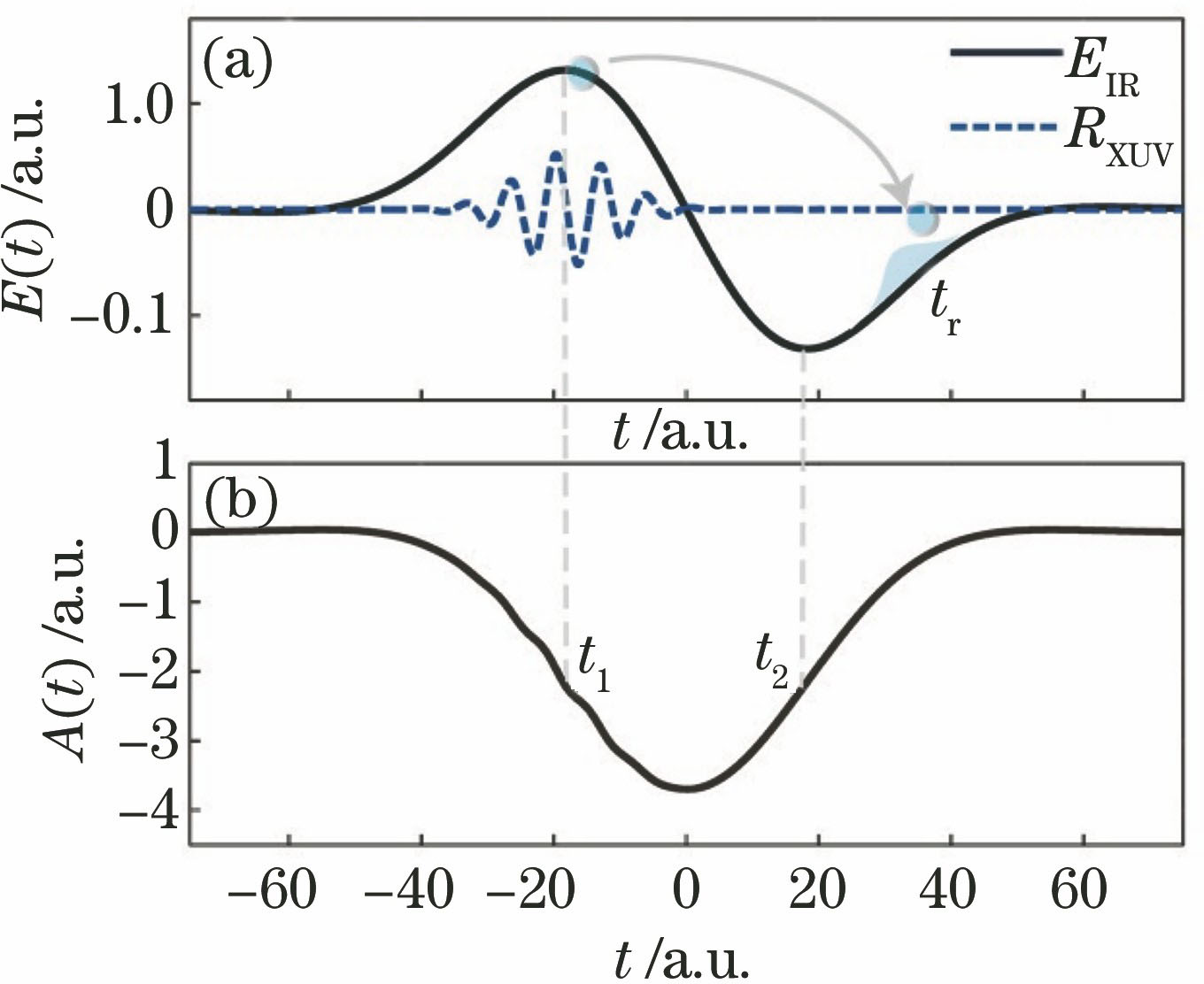
图 1.
Fig. 1. Combined laser fields as a function of time. (a) IR and EUV laser electric fields; (b) vector potential of the combined laser fields. The IR pulse has the wavelength 800 nm and the intensity I 1=1.5 × 1015 W/cm2. Time delay is t d=-17 a.u.
In the combined EUV and IR field, the double ionization undergoes the following several pathways. Firstly, the electron produced by the EUV field is driven back by the MIR field and rescatters with the He+ at

图 2.
Fig. 2. Correlated information of the electrons. (a) and (b) denote wave functions at time t =323 a.u. when the electron-electron repulsion is excluded or included, respectively; (c) momentum distribution of correlated double ionization in (a); (d) momentum distribution of correlated double ionization in (b); (e) and (f) denote the single electron momentum distribution after integrating the signals marked by the triangles in (c) and (d) along the vertical axis, respectively
3.2 Anticorrelated double ionization
Besides the distinct wave function distribution in the first quadrant in

图 3.
Fig. 3. Correlated information of the electrons. Photoelectron wave function in (a) space and (b) momentum representations at time t =344 a.u.; (c) electron-electron joint energy spectrum; (d) sum energy of two electrons obtained by integrating the signals in (c) along the lines E 1+E 2=const. The IR wavelength is 1200 nm, and the intensity I 1=8.3×1014 W/cm2. The time delay is

图 4.
Fig. 4. Ratio (anticorrelated double ionization probability to correlated double ionization probability) as a function of the screening parameter m ee. Other parameters for the line with open circles are the same as those used in Fig. 3 . For the line with crosses and line with stars, MIR intensities are I 1=5×1014 W/cm2 and 5.3×1014 W/cm2. MIR wavelengths are both 1500 n
We are still not sure how this energy sharing between the oppositely propagating electron pair works. One possible mechanism could be that the rescattering happens when both the electric field and vector potential are close to zero. During the rescattering, the kinetic energy of the freed electron is reallocated between two electrons. However, for a multicycle laser pulse, rescattering occurs when the temporary vector potential is maximum and the subsequent laser field will streak the two freed electrons and thus destroy the concentric arcs.
It is expected that Coulomb interaction between two electrons is important for the anticorrelated momentum distribution. To see how the electron-electron repulsion modifies the electron-electron joint momentum distribution, we screened the electron-electron repulsion by setting a screening factor
The ratio presented in
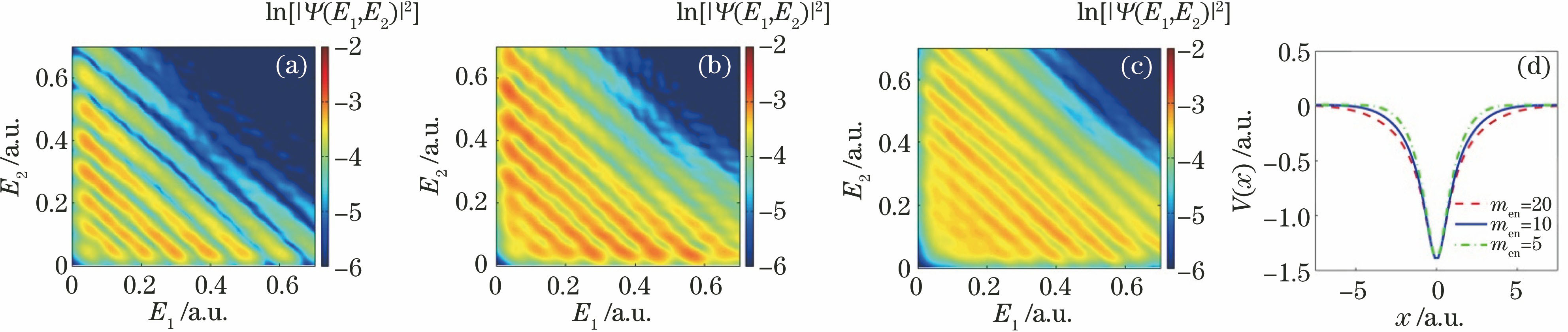
图 5.
Fig. 5. Anticorrelated joint electron-electron energy spectra. (a), (b) and (c) are joint electron-electron energy spectra for anticorrelated double ionization in the second and fourth quadrants at m en=20, 10, 5, respectively; (d) potentials at different screening parameters (m en=20, 10, 5). The MIR laser parameters are λ 1=2000 nm, I 1=3×1014 W/cm2. Time delay t
In addition to modifying the effective electron-electron repulsion, we also studied the influence of the Coulomb attraction between the electron and nucleus. In the potential described in Eq. (4), we screened the Coulomb attraction by modifying
4 Conclusion
In conclusion, the double ionization triggered by an EUV pulse and an extremely short MIR pulse is studied. For such an extremely short MIR pulse, the rescattering occurs at the tail of the laser pulse, and both freed electrons are not streaked after double ionization. Hence, the nonsequential and sequential double ionization wave packets may interfere with each other. Some slow electrons triggered by rescattering may propagate oppositely though they are strongly correlated. This study shows that the joint electron-electron momentum distribution depends on the Coulomb potentials and rescattering energies.
[5] Pedatzur O, Orenstein G, Serbinenko V, et al. Attosecond tunnelling interferometry[J]. Nature Physics, 2015, 11(10): 815-820.
[6] Keldysh L V. Ionization in the field of a strong electromagnetic wave[J]. Soviet Physics JETP, 1965, 20(5): 1307-1314.
[13] Corkum P B. Recollision physics[J]. Physics Today, 2011, 64(3): 36-41.
[16] Zhang W B, Gong X C, Li H, et al. Electron-nuclear correlated multiphoton-route to Rydberg fragments of molecules[J]. Nature Communications, 2019, 10: 757.
[18] Lein M. Gross E K U, Engel V. Intense-field double ionization of helium: identifying the mechanism[J]. Physical Review Letters, 2000, 85(22): 4707-4710.
[25] Shaaran T, Nygren M T. Figueira de Morisson Faria C. Laser-induced nonsequential double ionization at and above the recollision-excitation-tunneling threshold[J]. Physical Review A, 2010, 81(6): 063413.
[34] Lein M. Gross E K U, Engel V. Discrete peaks in above-threshold double-ionization spectra[J]. Physical Review A, 2001, 64(2): 023406.
[40] Press WH, Teukolsky SA, Vetterling WT, et al.Numerical recipes[M]. Cambridge: Cambridge University Press, 1992.
[41] Parker J S. Doherty B J S, Taylor K T, et al. High-energy cutoff in the spectrum of strong-field nonsequential double ionization[J]. Physical Review Letters, 2006, 96(13): 133001.
Article Outline
许亮, 吴婉阳, 何峰. 氦原子在超短中红外脉冲和极紫外光场作用下的双电离[J]. 中国激光, 2019, 46(5): 0508022. Liang Xu, Wanyang Wu, Feng He. Double Ionization of Helium in Extreme Ultraviolet Pulse in Presence of Extremely Short Mid-Infrared Pulse[J]. Chinese Journal of Lasers, 2019, 46(5): 0508022.