太阳上层过渡区极紫外三级次无狭缝成像光谱仪系统设计
Solar transition region is a highly dynamic plasma region with a temperature of 0.02-1 MK in the solar upper atmosphere, which is the key field of solar physics research. Extreme ultraviolet (EUV) multi-order slitless imaging spectrometer can observe the sun in a large field of view (FOV) simultaneously with high spectral, spatial, and temporal resolution. We propose and design a novel solar EUV slitless imaging spectrometer operating at Ne VII 46.52 nm. This instrument can simultaneously correct the off-axis grating aberrations of three diffraction orders in a large FOV (24′×24′) while meeting the requirements of light weight, compact volume, and large aperture. We hope that our three-order slitless spectral imaging strategy and system design can provide a technical scheme that can achieve continuous snapshot high-resolution spectral imaging observations of the upper transition region.
This instrument utilizes two toroidal uniform line-space (TULS) gratings and a toroidal mirror as the diffraction element and the reflective element respectively to realize a new three-order spectral dispersion structure. The ±1 order dispersion planes overlap with each other and are orthogonal to the 0-order imaging plane without dispersion. We build the paraxial optical model and calculate the initial solution of the optical system. By adopting the multi-configuration of ZEMAX software and the self-defined optimization function, the initial structural parameters of the three-order subsystems are globally optimized by considering the aberration correction conditions of the TULS grating, system resolution requirements, and geometric constraints. Finally, the tolerance analysis is carried out to obtain an optical system with reasonable tolerance allocation.
Figs. 6 (a)-(c) show the optical layout of the entire instrument, ±1 order subsystems, and 0-order subsystems respectively. This instrument consists of three hyperboloidal mirrors with off-axis aperture, three Si filters, two TULS gratings, a toroidal mirror, a field aperture, and three independent planar detectors. This entire instrument has a compact optical envelope volume of 950 mm×280 mm×240 mm, the focal length is 3200 mm, and the FOV is 24′×24′. Meanwhile, we employ a Si filter with a thickness of 0.1 μm to suppress the strong solar radiation in the visible band. The transmittance curve is shown in Fig. 7. The same periodic Sc/Si multilayers are coated at all optical surfaces to provide the reflectivity at 46.52 nm. The reflectivity curve is shown in Fig. 8. To reduce the conversion costs of the system, we add constraint conditions in the optimization process to make the final optimized TULS grating and mirror have identical surface height distribution and grating groove density. The surface height distribution is shown in Fig. 9.
Figs. 10 (a)-(c) are the RMS radius field map of the spot diagram of the three-order subsystems. Except for the marginal FOV of +1 order, the RMS radius of the spot diagram in the full FOV of each order is less than half of the pixel size. Figs. 11 (a)-(c) are the three-order modulation transfer function (MTF) curves respectively. Except for a few marginal FOV, all orders are greater than 0.6 at the Nyquist frequency (41.67 lp/mm), which is close to the diffraction limit. The image quality evaluation shows that the system has excellent imaging performance.
Figs. 12 (a)-(c) are the geometric ensquared energy curves of the instrument. Except for a few marginal FOV, the square full width with 80% ensquared energy at all orders is less than the pixel size. Considering the sampling frequency of the detector, the spatial resolution of the system is 1.547″. By adopting the theoretical calculation method, we obtain the pixel spectral resolution (Δλ) at -1 order and +1 order is 0.00622 nm and 0.00509 nm,respectively. The spot diagram distribution in the full FOV at ±1 order is shown in Figs. 13 (a)-(b). The spot diagram of three wavelengths with a wavelength difference Δλ is obviously separated on the image plane, which indicates that the spectral resolution of the system is 0.0078 nm.
Table 5 shows the tolerance limits of key components of the system. Under this tolerance, the RMS radius of the spot diagram on the image plane has a 90% probability of changing within 7.44 μm, and an 80% probability of changing within 6.4 μm. Tolerance analysis is carried out for the system with single grating working simultaneously at three orders. The results are shown in Table 5. The comparison shows that the designed instrument has a looser tolerance.
We propose and design a novel three-order slitless imaging spectrometer. A concave grating array is adopted as the diffractive optical element, and a single grating only works at a single diffraction order to correct the off-axis grating aberration in a large FOV. This system selects Ne VII 46.52 nm spectral line as the central wavelength, and it can realize high-resolution observation under a large FOV (24′×24′). The spatial resolution of the system is 1.547″, and the spectral resolution obtained by spectral data inversion is 0.0078 nm. The performance evaluation and tolerance analysis of the system show that the new imaging spectrometer has excellent imaging performance, compact system volume, and loose tolerance. Thus, it is suitable for deployment on remote-sensing satellite platforms to achieve high-resolution observation of solar upper-layer transition regions.
1 引言
太阳是迄今为止唯一能被研究者观测到其详细特征和物理过程的恒星[1]。通过空间太阳观测研究太阳上发生的各种物理过程,能够为研究宇宙中其他天体在不同物理参数下的活动特征提供重要参考[2]。空间太阳观测能为粒子物理学、等离子体物理学、磁流体力学等学科提供地面实验室无法获得的研究数据[3-4]。耀斑和日冕物质抛射等太阳爆发事件是灾害性空间天气的源头,通过研究太阳爆发事件引发灾害性空间天气的因果链,可以大大提升空间天气预报能力,进而减轻乃至避免其可能对高科技系统和人类生存环境造成的危害[5-7]。色球和日冕之间的过渡区域是一个受磁场控制的高度动态的等离子体薄层,普遍存在着各种小尺度的活动现象和精细结构[8]。过渡区域的研究被认为是建立太阳上层大气的物质循环和能量输运机制、解决日冕反常加热和太阳风起源两大难题的关键[9]。在0.02~1.00 MK的过渡区域温度下,处于不同电离态的离子会辐射出丰富的极紫外发射谱线,利用极紫外光谱学观测和诊断技术可以实现过渡区域等离子体特征的精确测量,进而为研究过渡区域的各种小尺度结构和瞬变事件提供数据支持[10]。
自20世纪70年代以来,多国开始以星载的极紫外光谱探测设备开展过渡区域的观测[11]。美国Skylab空间站上的S082A仪器[12]是一款摄影记录的无狭缝成像光谱仪,可在17.1~33.5 nm或32~63 nm波段同时实现多个极紫外谱线的全日面成像,空间分辨率为2″~10″,光谱分辨率最高可达0.0027 nm,因极紫外发射谱线分布密集,S082A仪器获得的相邻谱线的图像可能存在重叠,造成仪器的光谱分辨能力下降,仅能在少数孤立的强发射谱线如He II 30.4 nm、Ne VII 46.52 nm处取得良好的观测效果[11]。1995年,欧美联合发射的SOHO卫星[13]上搭载的日冕诊断光谱仪CDS[14-15],包含了正入射与掠入射光栅光谱仪两个通道,其中正入射通道的波段为31~38 nm与52~63 nm,空间分辨率约为6″,光谱分辨率为0.008 nm,单层镀膜的极紫外光栅的正入射反射率随波长的减小而迅速下降,该通道的波段受到限制;通过掠入射的光栅工作方式,掠入射通道实现了15~80 nm的宽波段覆盖,但该通道存在消像散能力不足的问题,进而限制了该通道的光谱分辨率(0.021 nm)。2022年,中国发射的SATech-01卫星上搭载的极紫外成像仪SUTRI[16],实现了上层过渡区域关键谱线Ne VII 46.52 nm窄波段全日面高分辨率成像,空间分辨率为8″,因极紫外成像仪普遍不具有高光谱分辨能力,SUTRI无法获得高分辨率光谱数据。日本计划于2026年发射的Solar-C卫星[17-18]上搭载的极紫外高效率光谱望远镜EUVST[19],其二级光谱区包括了46.3~54.2 nm波段,涵盖了Ne VII 46.52 nm重要谱线,预计最高空间分辨率可达0.4″,最高光谱分辨率为0.003 nm。
国内外长期的太阳物理学研究结果表明,上层过渡区域是太阳大气中温度在0.1~0.8 MK的等离子体区域,该温度范围是理解太阳大气物质和能量传输机制的关键,对诊断各类太阳爆发活动中的物理过程也具有重要意义[9]。Ne VII 46.52 nm谱线是上层过渡区域在45~48 nm波段的唯一强辐射谱线,利用该谱线开展上层过渡区域的高分辨率光谱学探测,获取温度约0.5 MK的上层过渡区域等离子体特征的精确信息,能够为建立太阳低层大气与日冕之间缺失的联系、研究太阳爆发事件的动力学与能量学提供关键信息[9,16]。SUTRI实现了Ne VII 46.52 nm谱线的全日面高分辨率成像观测,然而目前尚且缺少一款针对该谱线探测设计且能够在大视场范围内实现高分辨率快速二维光谱成像观测的极紫外仪器。鉴于此,本文设计了一款工作于Ne VII 46.52 nm窄波段的无狭缝成像光谱仪,仪器采用新的三级次无狭缝光谱色散结构,其中发生色散的±1级次色散平面相互重合,且与非色散的0级次成像面正交,单次快照可同时获得三幅大视场(24′×24′)极紫外窄波段(46.0~47.0 nm)的太阳图像。光谱成像质量评价和公差分析结果表明,该仪器能够实现大视场下的离轴光栅像差校正,各级次均取得良好的成像质量,且具有较大的公差容限,兼具轻量化、小体积、大口径的特点,适合卫星遥感平台的应用。
2 三级次无狭缝光谱成像概念
2.1 新型三级次无狭缝光谱成像架构
MOSES[20-21]的成功实践证明了无狭缝成像光谱仪能够获取传统仪器无法得到的研究太阳大气动态变化的关键数据[22]。然而,MOSES以及邢阳光等[23]设计的MOSES改进型仪器都存在一些缺陷,MOSES存在仪器体积过大、离轴光栅像差校正能力不足导致的视场受限、缺少中间像条件下形成的视场光阑可能造成的视场内谱线受到“污染”、增大光谱数据反演难度等弊端;邢阳光等设计的仪器改善了MOSES的部分缺陷,但也存在采用椭球面变线距(EVLS)光栅导致仪器工程化制造难度极大、采用单光栅同时工作于三个级次的仪器结构导致光栅公差容限要求极为严格等不足。
为解决现有无狭缝仪器存在的弊端,本文提出了一种新的三级次无狭缝成像光谱仪设计方案,采用两个超环面等线距(TULS)光栅作为分光元件可提供±1级次的衍射成像,而0级次的非色散成像由反射镜提供。如
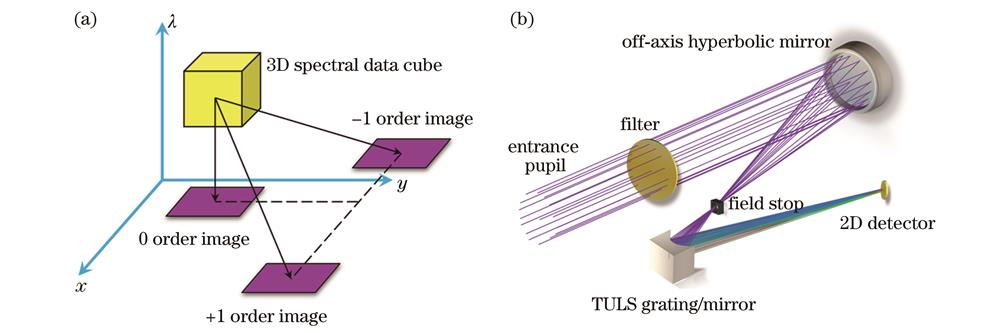
图 1. 新型三级次无狭缝光谱成像概念。(a)三级次无狭缝成像光谱仪的色散方向图解;(b)三级次无狭缝成像光谱仪的单个级次子系统示意图
Fig. 1. New concept of three-order slitless spectral imaging. (a) Dispersion direction diagram of three-order slitless imaging spectrometer; (b) schematic of single-order subsystem of three-order slitless imaging spectrometer
由于采用了单个光栅工作在一个衍射级次上的新型光谱色散架构,因此采用等线距的光栅即可实现大视场下该级次的离轴光栅像差校正,进而获取较大的仪器观测视场。另外,所采用的TULS光栅的加工与检测技术目前比较成熟,仪器的工程化制作极具可行性。三个级次的子系统仅通过视场光阑耦合,各子系统的光学元件相对独立,因此相比于单光栅三级次的系统具有更大的公差容限。具有新型光谱色散结构的三级次无狭缝成像光谱仪在各衍射级次均能取得优良的空间和光谱成像性能,兼具轻量化、小体积、大口径的应用需求,适合部署于卫星遥感平台。
2.2 Ne VII 46.52 nm谱线科学价值
如
![太阳大气温度随表面高度的变化曲线图[9]](/richHtml/gxxb/2024/44/6/0611001/img_02.jpg)
图 2. 太阳大气温度随表面高度的变化曲线图[9]
Fig. 2. Curve of solar atmospheric temperature changing with surface height[9]
2.3 系统技术指标
采用单光栅单级次的光谱色散结构可显著增强系统校正离轴光栅像差的能力,系统视场可以达到24′×24′。与MOSES[20](10′×20′)和邢阳光等设计的仪器[23](20′×20′)相比,本文设计的系统能够对更大的日面区域(全日面视场为32′)实现窄波段高分辨率观测。过渡区爆发事件(EEs)是过渡区重要的小尺度瞬变现象之一,其多普勒速度为50~200 km/s[8],根据EEs的光谱特征,系统像元光谱分辨率应优于0.0078 nm/pixel,反演的光谱分辨率应优于0.0078 nm。由SUMER[24]和CDS等取得的观测数据可知,EEs的空间尺度为1500~1800 km[10],因此本文设计的工作于517 km高度、98°倾角的太阳同步轨道的成像光谱仪的空间分辨率应优于2″(在近地轨道,1″对应约750 km的日面尺度)。系统总体设计指标如
表 1. 三级次无狭缝成像光谱仪的技术指标
Table 1. Technical specifications for three-order slitless imaging spectrometer
|
3 系统设计原理
3.1 TULS光栅像差校正条件
本文设计的系统采用的TULS光栅的像差校正原理图如
TULS光栅在光栅色散方向(子午方向)和垂直光栅色散方向(弧矢方向)内具有不同的曲率半径,可分别用子午半径R和弧矢半径ρ来表示,其光栅面型方程为
Haber[25]在1950年实现了TULS光栅的像差解析,经过Huber等[26]、Harada等[27]、Poletto等[28]、Yu等[29]的研究,目前TULS光栅的像差校正理论已相当成熟。鉴于此,本文直接给出了在扩展光源发散照明条件下TULS光栅主要像差(像散和离轴离焦)的校正条件[28]。
校正子午方向(光栅色散方向)的像散的条件为
校正弧矢方向(垂直于光栅色散方向)的像散的条件为
式(
离轴离焦像差的校正条件为
式中:β为光栅的横向放大率。本文设计的非罗兰圆结构的TULS光栅的衍射角和入射角在满足
3.2 初始解与评价函数
成像光谱仪在空间成像平面内的近轴光学模型如

图 4. 无狭缝成像光谱仪在空间成像平面内的近轴光学模型图
Fig. 4. Paraxial optical model diagram of slitless imaging spectrometer in spatial imaging plane
G为TULS光栅在空间成像面内的等效模型,焦距为fG,入射臂长为rA,出射臂长为rB,G的子午半径为R,弧矢半径为ρ,令R=ρ,则G简化为一个球面等线距(SULS)光栅。成像光谱仪系统的焦距为f,由近轴光学原理可知
成像光谱仪的初始结构应当满足
式中:e为探测器的像元尺寸。系统的像元光谱分辨率
式中:m为衍射级次;d0为光栅刻线间距;ψ为衍射光线在探测器上的入射角。在进行初始结构求解时,可忽略这两个角度的余弦值。
由高斯公式可知入射臂长rA和出射臂长rB在空间成像面内满足:
考虑到R=ρ的简化假设,则焦距fG=R/2=ρ/2。
成像光谱仪的初始结构还应当满足
式中:L为系统轴向长度;W为系统在±1级次色散平面内的尺寸;D为系统的入瞳直径。由于±1级次子系统位于同一色散平面内,为获得合理的结构布局,不同级次的主反射镜和探测器之间应避免产生空间干涉,初始结构参数需满足
式中:i+1、θ+1分别为+1级次光栅的入射角和衍射角;i-1、θ-1分别为-1级次光栅的入射角和衍射角;ω为离轴半视场角。
为获得系统初始解,需要确定一些已知量,包括轴向长度L、±1级次色散平面内的仪器尺寸W、光栅横向放大率β、光栅刻线间距d0、离轴半视场角ω。依次使用式(
表 2. 无狭缝成像光谱仪的初始结构参数
Table 2. Initial structural parameters of slitless imaging spectrometer
|
由计算获得的初始解并不是系统最优解,需要在ZEMAX软件中通过多重结构功能和像差优化函数[30],实现对系统三个级次像差的同时校正。像差优化函数可定义为
式中:λ0为极紫外窄波段的中心波长;φ(m)为各级次的权重因子,取相同值;τi(i=1,2,
4 光线追迹与性能评价
4.1 系统设计流程
为确保软件优化过程具有较高的收敛速度,依次使用式(
4.2 系统设计结果
最终优化得到的光学系统的技术指标和元件参数如
表 3. 三级次无狭缝成像光谱仪的技术指标和元件参数
Table 3. Specifications and optical element parameters for three-order slitless imaging spectrometer
|

图 6. 三级次无狭缝成像光谱仪光路原理图。(a)3D模型图;(b)±1级次子系统光路布局图;(c)0级次子系统光路布局图
Fig. 6. Optical layouts of three-order slitless imaging spectrometer. (a) 3D model diagram; (b) optical layout of ±1 order subsystems; (c) optical layout of 0-order subsystem
为了减少次镜的面型种类以降低仪器成本,在满足成像质量的前提下,通过加入约束条件使得三个级次的次镜面型相同,并且±1级次光栅刻线密度相同。最终优化得到的次镜面型矢高分布如
4.3 系统性能评价

图 10. 弥散斑RMS半径随二维视场变化的分布图。(a)-1级次;(b)0级次;(c)+1级次
Fig. 10. RMS radius of defocused spot varying with 2D field. (a) -1 order; (b) 0 order; (c) +1 order

图 11. 仪器在不同衍射级次下的MTF。(a)-1级次;(b)0级次;(c)+1级次
Fig. 11. MTF of design system under different diffraction orders. (a) -1 order; (b) 0 order; (c) +1 order
选用圈入弥散斑80%能量的方形全宽对应的空间分辨率作为评价系统空间分辨率的指标,
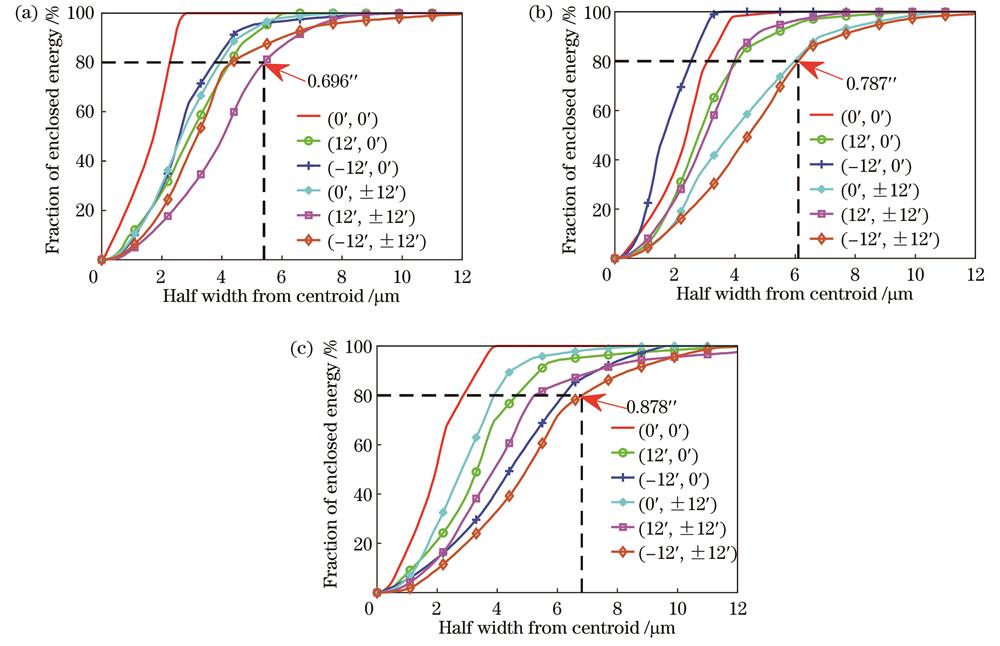
图 12. 用于评价光学系统空间分辨率的几何圈入能量。(a)-1级次;(b)0级次;(c)+1级次
Fig. 12. Geometric ensquared energy to evaluate system's spatial resolution. (a) -1 order; (b) 0 order; (c) +1 order
系统的光谱分辨率取决于TULS光栅在±1级次的设计结果,利用
表 4. 理论计算得到的±1级次光谱分辨率
Table 4. Theoretically calculated spectral resolution of ±1 order
|
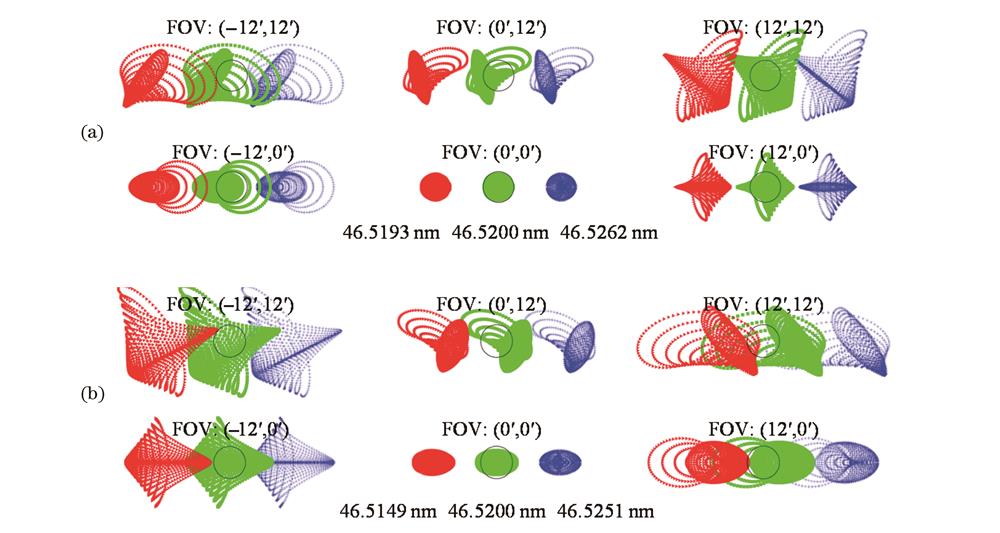
图 13. -1级次和+1级次的光谱分辨率验证。(a)-1级次;(b)+1级次
Fig. 13. Spectral resolution verification for -1 order and +1 order. (a) -1 order; (b) +1 order
4.4 公差分析
将制造公差和装配公差引入无狭缝成像光谱仪系统中,分析其对光谱成像性能的影响,并获取合理的公差分配。在ZEMAX软件中给定初始的公差范围,公差仿真过程中采用反灵敏度分析方法,将探测器作为后焦距补偿元件,采用像面弥散斑RMS半径作为评价标准,蒙特卡罗循环次数设置为1000次,通过反复迭代得到系统关键元件的公差容限如
表 5. 系统关键元件的公差容限
Table 5. Tolerance limits of key components of system
|
本文对单个TULS光栅同时工作于三个级次的光学系统进行了公差分析,得到的系统关键元件的公差容限如
5 结论
提出并设计了一款新型三级次无狭缝成像光谱仪,仪器采用两个TULS光栅作为衍射分光元件,提供±1级次的衍射成像,0级次的非色散成像由反射镜提供。系统在Ne VII 46.52 nm发射谱线下可实现24′×24′视场的高分辨率光谱成像观测,空间分辨率为1.547″,通过三个级次图像的光谱数据反演,可提取到分辨率为0.0078 nm的高光谱数据。系统性能评价和公差分析结果表明新型成像光谱仪具有优良的成像质量、紧凑的系统体积、宽松的公差容限。为了实现对太阳上层过渡区爆发活动的高时空分辨观测,在仪器的实际工程化研制的过程中,高精度且快速收敛的光谱数据反演算法是必需的;另外,为了满足基于遥感卫星平台的应用,仪器的平场及辐射定标,杂散光分析、抑制,以及热流控制等是不可忽略的关键技术。
[1] 刘睿, 陈耀, 邓元勇, 等. 中国太阳物理学研究进展[J]. 科学通报, 2019, 64(19): 2011-2024.
Liu R, Chen Y, Deng Y Y, et al. Recent research progress of solar physics in China[J]. Chinese Science Bulletin, 2019, 64(19): 2011-2024.
[2] 颜毅华, 谭宝林. 太阳物理研究与发展[J]. 中国科学院院刊, 2012, 27(1): 59-66.
Yan Y H, Tan B L. Research and development of solar physics[J]. Bulletin of Chinese Academy of Sciences, 2012, 27(1): 59-66.
[3] 杨孟飞, 代树武, 王颖, 等. 太阳空间探测进展与展望[J]. 中国空间科学技术, 2022, 42(5): 1-10.
Yang M F, Dai S W, Wang Y, et al. Progress and prospect of solar exploration in space[J]. Chinese Space Science and Technology, 2022, 42(5): 1-10.
[4] 陈鹏飞. 对太阳物理发展趋势的展望[J]. 中国科学: 物理学 力学 天文学, 2021, 51(11): 140-144.
Chen P F. Perspectives on the promotion of solar physics[J]. Scientia Sinica: Physica, Mechanica & Astronomica, 2021, 51(11): 140-144.
[5] 方成. 走进我们生活的新学科: 空间天气学[J]. 自然杂志, 2006, 28(4): 194-198, 250.
Fang C. Space weather comes into our life[J]. Chinese Journal of Nature, 2006, 28(4): 194-198, 250.
[6] 魏奉思. 空间天气科学与有效和平利用空间[J]. 航天器环境工程, 2014, 31(5): 457-463.
Wei F S. Space weather science and the effective peaceful use of space[J]. Spacecraft Environment Engineering, 2014, 31(5): 457-463.
[7] 王水. 空间天气研究的主要科学问题[J]. 中国科学技术大学学报, 2007, 37(8): 807-812.
Wang S. Major science problems on space weather research[J]. Journal of University of Science and Technology of China, 2007, 37(8): 807-812.
[8] 田晖, 谭博, 夏利东, 等. 太阳过渡区的结构与特性[J]. 天文学进展, 2009, 27(1): 1-13.
Tian H, Tan B, Xia L D, et al. Structure and characteristics of the solar transition region[J]. Progress in Astronomy, 2009, 27(1): 1-13.
[9] Tian H. Probing the solar transition region: current status and future perspectives[J]. Research in Astronomy and Astrophysics, 2017, 17(11): 110.
[10] 章敏, 夏利东, 黄正化. 太阳过渡区爆发事件的观测及理论研究进展[J]. 天文学进展, 2010, 28(3): 229-242.
Zhang M, Xia L D, Huang Z H. Observational and theoretical studies on explosive events in the solar transition region[J]. Progress in Astronomy, 2010, 28(3): 229-242.
[11] 白先勇, 田晖, 邓元勇, 等. 太阳极紫外光谱探测的历史与展望[J]. 空间科学学报, 2023, 43(3): 406-422.
Bai X Y, Tian H, Deng Y Y, et al. Current status and future perspectives of solar spectroscopic observations at extreme ultraviolet wavelengths[J]. Chinese Journal of Space Science, 2023, 43(3): 406-422.
[12] Tousey R, Bartoe J D F, Brueckner G E, et al. Extreme ultraviolet spectroheliograph ATM experiment S082A[J]. Applied Optics, 1977, 16(4): 870-878.
[13] Domingo V, Fleck B, Poland A I. The SOHO mission: an overview[J]. Solar Physics, 1995, 162(1): 1-37.
[14] Harrison R A, Sawyer E C, Carter M K, et al. The Coronal Diagnostic Spectrometer for the solar and heliospheric observatory[J]. Solar Physics, 1995, 162(1): 233-290.
[15] Lidiard K A, Gray P F. Optical design of the coronal diagnostic spectrometer (an instrument on the Solar and Heliospheric Observatory)[J]. Optical Engineering, 1997, 36(8): 2311-2319.
[16] Bai X Y, Tian H, Deng Y Y, et al. The solar upper transition region imager (SUTRI) onboard the SATech-01 satellite[J]. Research in Astronomy and Astrophysics, 2023, 23(6): 065014.
[17] Shimizu T, Imada S, Kawate T, et al. The solar-C_EUVST mission[J]. Proceedings of SPIE, 2019, 11118: 1111807.
[18] Shimizu T, Imada S, Kawate T, et al. The Solar-C (EUVST) mission: the latest status[J]. Proceedings of SPIE, 2020, 11444: 114440N.
[19] Suematsu Y, Shimizu T, Hara H, et al. Instrumental design of the Solar Observing Satellite: solar-C_EUVST[J]. Proceedings of SPIE, 2021, 11852: 118523K.
[20] Kankelborg C C, Thomas R J. Simultaneous imaging and spectroscopy of the solar atmosphere: advantages and challenges of a 3-order slitless spectrograph[J]. Proceedings of SPIE, 2001, 4498: 16-26.
[21] Fox J L, Kankelborg C C, Thomas R J. A transition region explosive event observed in he ii with the Moses sounding rocket[J]. The Astrophysical Journal Letters, 2010, 719(2): 1132-1143.
[22] 彭吉龙. 空间太阳极紫外无狭缝光谱成像技术综述[J]. 航天器环境工程, 2018, 35(5): 450-456.
Peng J L. Review of slitless spectral imaging technologies for space solar extreme-ultraviolet observation[J]. Spacecraft Environment Engineering, 2018, 35(5): 450-456.
[23] 邢阳光, 李林, 彭吉龙, 等. 三级次太阳极紫外窄波段无狭缝成像光谱仪光学设计[J]. 光学学报, 2021, 41(7): 0730003.
[24] Wilhelm K, Curdt W, Marsch E, et al. SUMER - solar ultraviolet measurements of emitted radiation[J]. Solar Physics, 1995, 162(1/2): 189-231.
[25] Haber H. The torus grating[J]. Journal of the Optical Society of America, 1950, 40(3): 153-165.
[26] Huber M C E, Tondello G. Stigmatic performance of an EUV spectrograph with a single toroidal grating[J]. Applied Optics, 1979, 18(23): 3948-3953.
[27] Harada T, Sakuma H, Takahashi K, et al. Design of a high-resolution extreme-ultraviolet imaging spectrometer with aberration-corrected concave gratings[J]. Applied Optics, 1998, 37(28): 6803-6810.
[28] Poletto L, Thomas R J. Stigmatic spectrometers for extended sources: design with toroidal varied-line-space gratings[J]. Applied Optics, 2004, 43(10): 2029-2038.
[29] Yu L, Wang S R, Qu Y, et al. Broadband FUV imaging spectrometer: advanced design with a single toroidal uniform-line-space grating[J]. Applied Optics, 2011, 50(22): 4468-4477.
[30] Zeng J, Bayanheshig, Li W H, et al. Merit function to design holographic gratings for moderate-resolution monochromators[J]. Applied Optics, 2011, 50(33): 6179-6183.
[31] 彭吉龙, 朱光武, 韦飞, 等. 太阳极紫外多波段成像仪[J]. 空间科学学报, 2009, 29(4): 417-421.
Peng J L, Zhu G W, Wei F, et al. Solar extreme ultraviolet multichannel imager[J]. Chinese Journal of Space Science, 2009, 29(4): 417-421.
[32] 齐润泽, 张锦龙, 吴佳莉, 等. 极紫外-真空紫外薄膜光学元件研究[J]. 光学学报, 2022, 42(11): 1134003.
Article Outline
沈文杰, 邢阳光, 黄一帆, 彭吉龙, 代树武, 王颖, 朱成林, 闫雷, 刘越, 李林. 太阳上层过渡区极紫外三级次无狭缝成像光谱仪系统设计[J]. 光学学报, 2024, 44(6): 0611001. Wenjie Shen, Yangguang Xing, Yifan Huang, Jilong Peng, Shuwu Dai, Ying Wang, Chenglin Zhu, Lei Yan, Yue Liu, Lin Li. System Design of Extreme Ultraviolet Three-Order Slitless Imaging Spectrometer for Solar Upper Transition Region[J]. Acta Optica Sinica, 2024, 44(6): 0611001.