Strong optical force of a molecule enabled by the plasmonic nanogap hot spot in a tip-enhanced Raman spectroscopy system
Download: 633次
1. INTRODUCTION
Light carries energy and momentum besides electromagnetic field. Therefore, light–matter interaction naturally brings about mechanical effects, among which the optical force occurring due to the exchange of momentum between light and matter is a prominent example. Optical force has been extensively explored in the optical tweezers system for capturing microscopic objects such as cells, micrometer beads, and nanoparticles. Since Ashkin first demonstrated the concept of negative light pressure due to the gradient force [1], the single-beam optical tweezers, which use a microscopic object to tightly focus a single laser beam, have become an extremely important tool in diversified fields of atomic spectroscopy, cell biology, and nano-biophotonics [2
Recently, many studies have combined optical tweezers and the concepts and techniques of near-field optics and nanophotonics, and successfully pushed optical tweezers into the nanoscale region. These near-field optical tweezers make the stable trapping of nanometer-size objects in the 10–100 nm range no longer a great challenge [10–
In the above plasmonic optical tweezers, the hot spot, with greatly enhanced optical field intensity in a nanoscale spatial region with a size far smaller than the wavelength of light, plays an important role in achieving the unique features of optical force. It is generally recognized that the plasmonic nanogap in the tip-enhanced Raman scattering (TERS) system, which is formed by a metallic tip (with a nanoscale curvature at the apex) sitting on a metallic thin film with controllable nanometer-size gap, provides a very unique hot spot [2123" target="_self" style="display: inline;">–
In this work, we theoretically explore the mechanical effect of this nanoscale localized hot spot acting upon the molecule embedded within the nanogap of the TERS system. We carry out a systematical numerical analysis based on a three-dimensional finite-difference time-domain (3D FDTD) calculation against the optical field distribution of this nanogap plasmonic hot spot, not only the field intensity but also the field gradient. Then we calculate the resulting gradient force upon the molecule by Eq. (
2. RESULTS AND DISCUSSION
The geometry model of a typical TERS system as studied in this work is illustrated in Fig.

Fig. 1. (a) Schematic diagram for a typical TERS system involving a molecule immersed within a mode that is excited by an incident p-polarized laser. (b) Schematic diagram showing the molecule affected by the gradient force coming from the highly localized and enhanced electric field hot spot within the nanogap.
To solve the optical force exerted on the molecule, the first step is to calculate the optical field around the molecule within the nanogap under the excitation of the incident laser, which is set to have an amplitude of . We used the 3D FDTD approach to numerically solve the optical field. In our 3D FDTD simulations, a cubic simulation region with the size of was used throughout all calculations. Perfectly matched layers boundary conditions were used on all boundaries of simulation region to avoid disturbance of the boundary reflection. In order to accurately simulate the 2 nm tip–substrate distance, the minimum uniform mesh size was set to 0.2 nm. The optical constant for silver was taken from Ref. [26]. The 3D FDTD approach allows us to determine precisely the 3D optical electric field distribution, including its intensity and vectorial direction, within and around the nanogap, where the molecule is located.
The next step is to determine the mechanical response, namely, the optical force of the molecule against the optical field within which it is immersed. In our work, the excited molecule was modeled using an electric dipole with a dielectric constant of 3, which is close to the value of the solid material made from the aggregation of these molecules as building block. Now we need to first estimate the linear polarizability of the molecule. We first consider a single organic molecule, which was systematically studied in Ref. [24]. This molecule exhibits a disk-like geometry, has a diameter of and thickness of . In this work, we assume that it could be modeled as a spherical molecule with a radius of and a dielectric coefficient . The linear molecular polarizability of this molecule could be predicted by the Clausius–Mossotti relationship and write The result is [23].
Generally, if the molecule size is much smaller than the laser wavelength (with radius less than ), we assume that the external electric field does not vary within the molecule. Therefore, the molecules are often regarded as point electric dipoles to account for their optical and mechanical responses [2729" target="_self" style="display: inline;">–
Having clarified the major contribution of gradient force upon the molecule, it is then straightforward to proceed to evaluate the optical force from the nanogap plasmon hot spot numerically and theoretically. In our simulations, we have solved the electric field distribution and then calculated the optical force upon the molecule by using Eqs. (
For a dielectric particle, the gradient force essentially depends on the local electric field distribution (in particular the field gradient) and the charge density distribution (in particular the effective dipole moment) induced in the particle. In TERS, the key contribution to the optical force is the highly localized field of hot spot associated with the strong LSPR with the plasmonic nanogap. Remarkably, this hot spot has a full width at half-maximum (FWHM) that is far smaller than the wavelength of light and readily reaches the nanometer scale regime. Compared with the diffraction-limited focusing of a Gaussian beam in ordinary single-beam microscopic-objective optical tweezers, the much sharper focusing of the plasmonic wave should be able to produce a stronger gradient force.
It is worthwhile to make a comparison with the more common and popular single-beam tweezers. As shown in Fig.

Fig. 2. (a) Schematic diagram of classical optical tweezers formed by a tightly focused Gaussian laser beam trapping a molecule. (b) Calculated optical gradient forces component in the -axis, -axis, and -axis directions in the plane of the focus point.
It has been well established that for optical tweezers, in principle a tiny focus spot of the trapping beam is necessary for providing a sufficient strength of gradient force to capture a molecule. For this reason, we turn to the TERS system for solution because the very tiny hot spot of local electric field enhanced by LSPR in the plasmonic nanogap should provide a much stronger gradient field. We study the distribution of gradient force and calculate the accurate value to provide a reliable analysis on whether the hot spot can trap the molecule.
In previous studies of TERS systems, the materials of the tip and substrate are generally gold or silver materials. Which material is better for studying optical forces in a TERS system? We know that the gradient force is related to the strength of the electric field. Thus we study the plane () and plane electromagnetic field distribution of silver and gold as the tip and substrate under the same conditions as shown in Figs.

Fig. 3. (a), (b) The plane ( ) and plane electromagnetic field distribution of an Ag tip (curvature radius of 6.25 nm). (c), (d) The plane ( ) and plane electromagnetic field distribution of an Au tip (curvature radius of 6.25 nm). (e), (f) The plane ( ) and plane electromagnetic field distribution of an Ag tip (curvature radius of 25 nm).
Since the incident light contains an electric field component in the -axis direction, it can excite the nanogap mode between the tip and the substrate. We use the 3D FDTD method to calculate the local electric field intensity. The normalized optical intensity of the local field against the incident light intensity (with amplitude ) within the plane passing the nanogap center, namely, , is displayed in Fig.
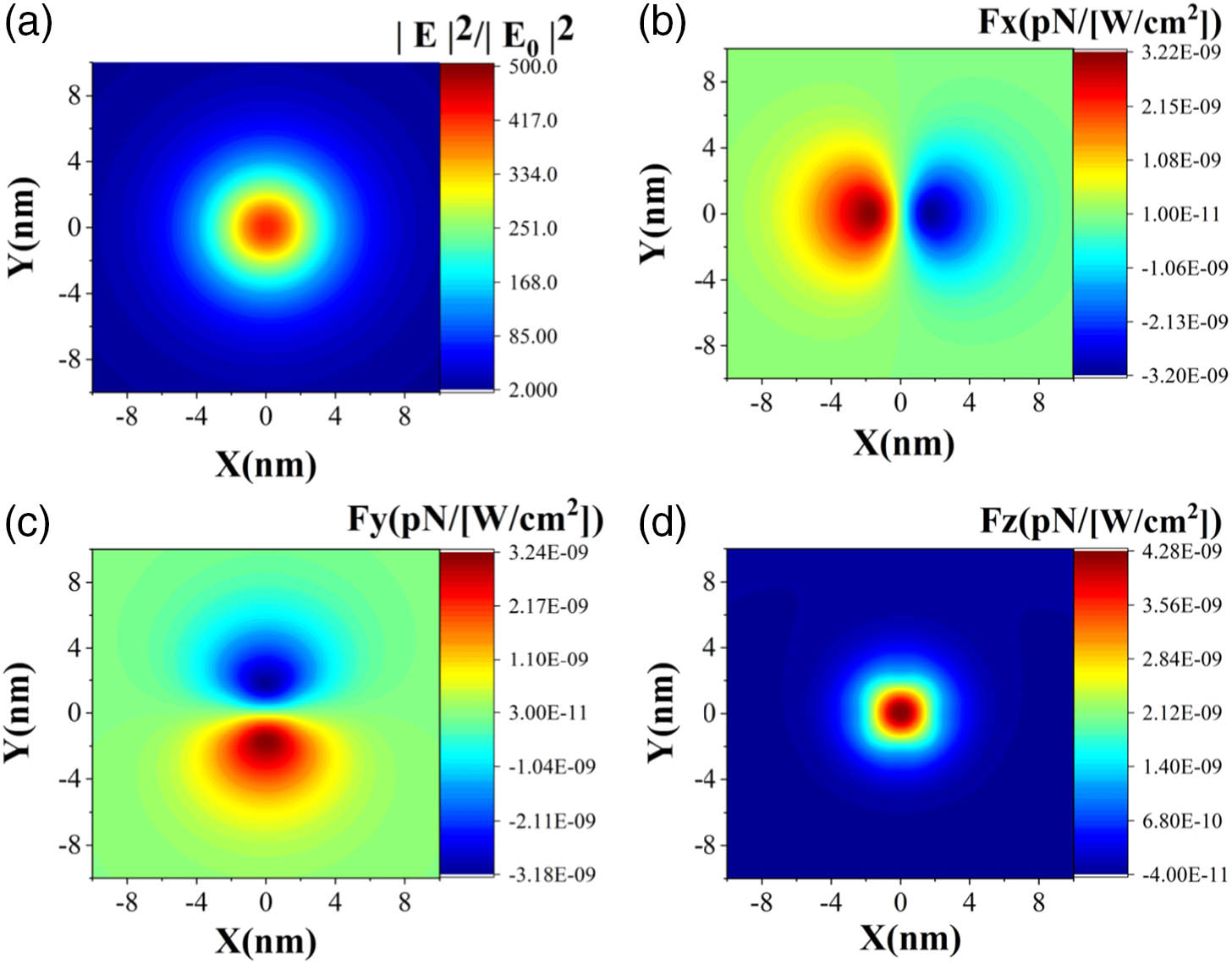
Fig. 4. Electric field and gradient force distribution in the plane located 1 nm below the Ag tip. (a) Electric field intensity distribution. Optical gradient force distribution for (b) -axis, (c) -axis, and (d) -axis components.
According to the above calculation results, the force of a single molecule in the nanogap plasmonic hot spot of the TERS system is similar to the situation of single laser beam optical tweezers. Yet, whether the molecule can be stably captured to the center of this hot spot depends on the longitudinal force and the radial force. The radial force traps the molecule toward the center of the nanogap, as shown in Fig.
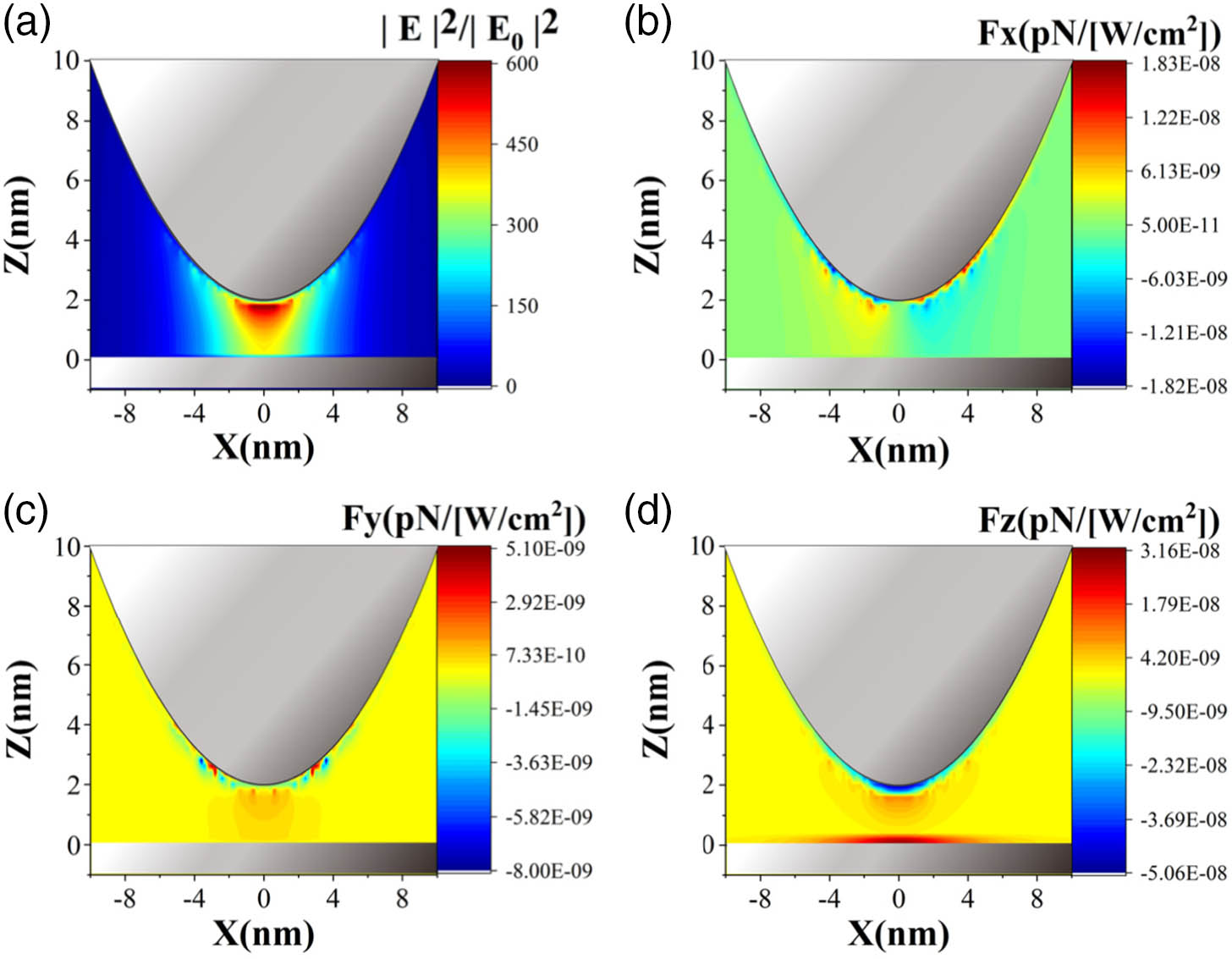
Fig. 5. plane field and gradient force distribution of the TERS system. (a) Electric field intensity distribution. Optical gradient force distribution for (b) -axis, (c) -axis, and (d) -axis components.

Fig. 6. plane field and gradient force distribution of the TERS system. (a) Electric field intensity distribution. Optical gradient force distribution for (b) -axis, (c) -axis, and (d) -axis components.
As shown in Figs.

Fig. 7. (a) One-dimensional distribution of the gradient force of the molecule along the direction of the hot spot. One-dimensional gradient force distribution in the (b) and (c) directions of the hot-spot center ( ).
As a result, we believe that there exists a stable region of optical trapping in the longitudinal force of the molecule in this gap, rather than a single stable point in the usual situation of optical tweezers, as shown in Figs.
To see whether this stable optical trapping region is a stable 3D optical trap for the molecule to be tightly captured, we examine the gradient force distribution in the plane intersecting with a point () in this longitudinal stable region. In particular, we display the 1D gradient force curve along the -axis and -axis, which is the radial force, in Figs.
We proceed to examine the quantity of the hot-spot optical trap in reference to the conventional single-beam optical tweezers, which can be well described by the optical trap stiffness. The optical trap stiffness, defined by the amount of change in the optical trap force right at the center of the optical trap against a unit distance of deviation, is an important parameter for describing the stability of the optical trap. We find the stiffness of single-beam tweezers is about ] (the horizontal plane), as calculated from Fig.
Note that in our above calculations, we have taken a point-dipole model, where the molecule is modeled as a point dipole without geometric size but only with a certain size of electric polarizability. We proceed to consider a much smaller molecule, such as a (with the radius of 0.2 nm) molecule placed in this 2 nm nanogap to study the longitudinal force. The point-dipole model becomes more accurate for this small molecule. Figure

Fig. 8. One-dimensional distribution of the gradient force of a molecule along the directions of the hot spot; (a) curvature radius of 6.25 nm, (b) curvature radius of 25 nm.
3. CONCLUSIONS
In summary, we have theoretically studied and analyzed the optical force of a molecule embedded within the nanoscale hot spot of the TERS system. Usually, this system is dominantly used as a powerful means to enhance, detect, and image the Raman scattering signal of a molecule. The localized surface plasmonic resonance within the nanogap between the TERS tip and substrate will induce a significant local electric field enhancement in the nanoscale hot spot. The major purpose of this work is to explore other physical effects that might be involved with this nanoscale hot spot in addition to the well-established Raman scattering of the molecule. The optical force comes into this scope of physical insight and landscape of physical picture.
For this purpose, we have systematically investigated the local electric field and field gradient of the nanogap plasmon hot spot in 3D space within and around the nanogap via 3D FDTD simulations, and made a careful analysis over their overall distribution pattern, from which the optical gradient force upon the molecule can be calculated and analyzed. We have found that, due to the highly localized electric field, a true 3D optical trap can form at the hot spot, which allows the molecule to be trapped in the hot spot. Moreover, the optical energy density and optical force acting on a molecule can be greatly enhanced to a level far exceeding the conventional single laser beam optical tweezers. Our calculation results have shown that the stiffness of the hot-spot trap can reach a high value of about ] (the horizontal plane) and ] (the vertical axis direction), which is far larger than the stiffness of single-beam tweezers, ] (the horizontal plane). This hard-stiffness will enable the molecules to be stably captured in the plasmon hot spot. Our results indicate that TERS can become a promising tool of optical tweezers for trapping a microscopic object like molecules and doing Raman spectroscopic imaging and analysis at the same time. In addition, we expect the current work can open up a new avenue to deeply explore the multiphysics processes and effects that can exist and act simultaneously in the unique nanoscale hot spot offered by the TERS plasmonic nanogap. In some means, the well-established fluorescence and Raman scattering processes, the relatively less studied Rayleigh scattering process, and the optical force effect studied in this work are just some examples. This suggests that one can explore, examine, and analyze more deeply and systematically various optical, electronic, chemical, and mechanical processes and effects acting upon a molecule located within such a plasmonic nanogap. The results surely will greatly expand and deepen our understanding, knowledge, and insight on light–matter interaction at nanoscale and even atomic scale in these plasmonic nanostructures.
[3] D. G. Grier. A revolution in optical manipulation. Nature, 2003, 424: 810-816.
[8]
[9] D. G. Grier. A revolution in optical manipulation. Nature, 2003, 424: 810-816.
[30] J. P. Gordon. Radiation forces and momenta in dielectric media. Phys. Rev. A, 1973, 8: 14-21.
Article Outline
Li Long, Jianfeng Chen, Huakang Yu, Zhi-Yuan Li. Strong optical force of a molecule enabled by the plasmonic nanogap hot spot in a tip-enhanced Raman spectroscopy system[J]. Photonics Research, 2020, 8(10): 10001573.