Design and analysis of extended depth of focus metalenses for achromatic computational imaging
Download: 749次
1. INTRODUCTION
Over the last decade, image sensors have undergone dramatic miniaturization, thanks to advances in optical packaging and semiconductor-based photodetector technology. Even further size reduction, however, is required for emerging areas of machine vision, autonomous transportation, and augmented reality visors [13" target="_self" style="display: inline;">–
To eliminate higher-order diffraction, subwavelength diffractive optics, also known as metasurfaces, can be used [5]. Metasurfaces are two-dimensional optical elements consisting of quasi-periodic subwavelength resonators that are capable of abruptly introducing phase shifts onto a wavefront, enabling ultrathin optics and lenses [5
Another technique for mitigating chromatic aberrations is to employ freeform metasurfaces and computational imaging [34
Recently, full-color imaging in the visible wavelength regime was demonstrated using an EDOF metasurface and post-processing deconvolution [35]. Here, a rectangularly separable cubic phase mask (CPM) [45] was added to the standard hyperboloidal metalens phase, generating a non-rotationally symmetric extended focal spot. The longitudinally extended nature of the focal spots at different wavelengths is sufficient to compensate for the chromatic shift in the focal length. The EDOF property of the CPM enables the imaging system to capture useful spatial frequency information of the colored image so that computational reconstruction is possible [35]. The CPM is limited, however, in that it produces a transversely asymmetric point spread function (PSF) that makes imaging sensitive to the orientation of the element, often manifesting as asymmetric artifacts even after deconvolution. Additionally, the CPM produces a lateral shift of the PSF with a change in wavelength (due to creation of an accelerating Airy beam), which can contribute to distortions in imaging. One potential solution to this limitation is to utilize a rotationally symmetric PSF. Although there are previous works on symmetric, EDOF refractive optics using log-aspheres [46] and axicon-based lenses [47], they have not been used for correcting the strong chromatic aberrations encountered in a metasurface. In this paper, we extend the family of EDOF metasurfaces beyond a simple CPM. We design and fabricate four different types of EDOF metasurface lenses operating in the visible regime, including both rotationally symmetric and asymmetric phase profiles. We characterize the modulation transfer function (MTF) for all these lenses and demonstrate full-color imaging. A comparative analysis of all these lenses is also provided, evaluating in terms of optical bandwidth and image quality. All our EDOF metasurfaces demonstrate at least an order of magnitude larger optical bandwidth compared to a standard metalens. Full-color imaging in the visible range is achieved using all the EDOF lenses, outperforming the traditional metalens in terms of chromatic aberrations.
2. METHODS
An imaging system behaves as a linear system that maps the incoming light from a scene to the sensor. This mapping function is modeled by an object’s convolution with the element’s PSF. For imaging under incoherent light, the system provides a linear mapping from the input intensity to the output intensity, which is captured on the sensor array. By scanning a point source throughout the object volume and measuring the resultant intensity across the image volume, a 3D intensity impulse response, or PSF, can be measured. This method can fully characterize the imaging function of the system. Assuming the PSF is shift-invariant, we can treat the PSF as a kernel that convolves with the input to produce an image on the sensor plane. The PSF of an ideal lens is an Airy disk that, when the aperture is large, resembles a point, which enables capturing an almost exact replica of the scene. When the PSF deviates from a point, the captured image becomes blurry; however, with a PSF known a priori, an in-focus image can be retrieved via post-capture deconvolution if sufficient spatial frequency information at a high enough signal-to-noise ratio (SNR) is collected by the sensor. This information retrievability through computational imaging can be expressed in terms of the MTF of the optical element, which is given by the magnitude of the Fourier transform of the PSF. A broad MTF, one that does not drop to zero rapidly, signifies that a wide range of spatial frequencies from the object plane is captured at the sensor plane, corresponding to a PSF with a small spot size. On the other hand, a narrow MTF, one that decays rapidly, captures only a limited range of spatial frequency content, which precludes the possibility of computational reconstruction due to the zeros in the spatial frequency spectrum. A conventional, in-focus metalens exhibits a broad MTF when imaging with narrowband light, resulting in high-quality images. When imaging with a different wavelength at the same sensor plane, however, the spatial bandwidth of the MTF drastically decreases and the collected spatial frequencies from the scene are attenuated or eliminated. As some of the spatial frequencies are not collected, this results in an uncorrectable blur. With EDOF lenses, we can realize a similar PSF at the sensor plane for a broad and continuous range of wavelengths [48]. Moreover, the resulting MTF can capture a wider range of spatial frequencies, which is key to generating full-color images at high resolution.
Here, we designed four different EDOF lenses, namely cubic [45], shifted axicon, log-asphere [46], and SQUBIC [49] lenses. All, except for the cubic, are axially symmetric. We fix the aperture for each of these metasurfaces at 200 μm and select a nominal focal length of 200 μm, making the NA close to 0.45 for all designs. We emphasize that the small aperture of the metalens is due to the prohibitive cost and time for fabricating large-aperture lenses based on electron-beam lithography, and not due to the same scaling limitations as encountered with dispersion-engineered metalenses. At the time of writing, the highest demonstrated NA with a dispersion-engineered metalens is 0.35, the diameter of which was only 30 μm [50,51]. The cubic metalens utilizes a focusing phase mask combined with a cubic term to produce an MTF insensitive to wavelength [35], and the phase mask is where denotes the operating wavelength, and are the coordinates in plane, is the nominal focal length, represents the strength of the cubic term, and represents the radius of the phase mask. We chose , , , and for the design of the cubic metasurface. To compare the performance of the EDOF metasurfaces against the standard singlet metalens, we include a design with that imparts no cubic term to the wavefront. The log-asphere phase mask was inspired by a prior work [46] that divides the phase mask into annular zones with continuously varying focal lengths. The central annular zone has a focal length of and the outermost annular zone has a focal length of . This design effectively extends the focal length from to . The log-asphere phase mask is governed by the relation where and is the aperture radius of the phase mask. The parameter changes the intensity distribution over the optical axis. For the log-asphere phase mask, we set , making the intensity distribution uniform across the line of foci. The parameters we chose for the log-asphere metasurface design are , , , and . Similar to the log-asphere phase mask, the shifted axicon phase mask takes the same form as Eq. (
The SQUBIC metasurface was reported to have EDOF properties [49]. The phase mask directs a collimated light beam into a line of discrete foci, achieving EDOF, and is given by the equation where , given that NA is the nominal numerical aperture of the optics. Here, is a design parameter that determines the strength of the phase mask [49]. In our design, we set and . Figure
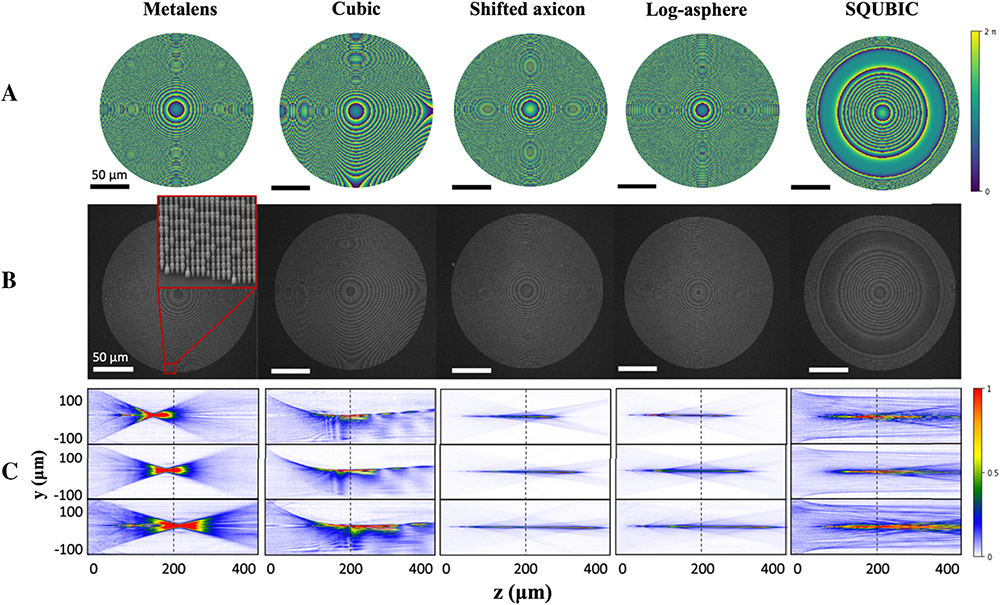
Fig. 1. EDOF metasurface design and measurements. (A) The phase masks of an ordinary metalens and four different EDOF metasurfaces. (B) Scanning electron micrographs of the fabricated metasurfaces. Inset shows the pillar distribution. (C) We experimentally measured the intensity along the optical axis where panels from top to bottom represent illumination by 625 nm, 530 nm, and 455 nm wavelengths. A cross section on the y –z plane is taken for each of the 3D PSFs.
These EDOF lenses are then implemented using cylindrical nanopillars [5,53] to ensure polarization insensitivity. These nanopillars are arranged on a square lattice. By varying the diameters of these nanoposts, the transmission coefficient imparted on incident light is modified as the coupling to and among different supported modes by the nanoposts changes, resulting in different phase shifts (Fig.
3. EXPERIMENT
A fiber-coupled LED light source (Thorlabs M625F2, M530F2, M455F1) is used to illuminate the fabricated metasurfaces (one ordinary metalens and four EDOF metasurfaces). A custom microscope mounted on a computer-controlled translation stage is used to take snapshots of the imaging plane. The focal length of the metalens is found to be 231 μm with the green LED. This deviation from the designed focal length (200 μm) could be due to the difference in the designed wavelength (550 nm) and the experimental light source wavelength (530 nm). The imperfect fabrication could also contribute to this deviation. The nominal focal length is thereafter set to 231 μm for all metasurfaces to accommodate this shift. To verify the EDOF, PSFs in different x–y planes are measured along the optical axis (-axis) starting from the metasurface () to to construct a 3D PSF [y–z plane is shown in Fig.

Fig. 2. Characterization of the metasurfaces. The PSFs of the singlet metasurfaces were measured under (A) 455 nm blue, (B) 530 nm green, and (C) 625 nm red. The corresponding x –y plane cross sections of the experimental MTFs are displayed with red lines from its PSF measured under red light, green lines under green light, and blue lines under blue light. (D) The x –y plane cross sections of the theoretical MTFs are displayed in row (E). The scale bar signifies 25 μm. The MTF plots have a spatial frequency normalized to 560 cycles/mm.
Table 1. Imaging Bandwidth, Defined as the Bandwidth at One Half of the Maximum PSF Similarity Coefficient
|
Our EDOF imaging system presents a problem in the form of , in which is the observed image, is the PSF or blur kernel, is the latent image, and is noise that corrupts the captured data. Although several methods exist to restore the image given and , we chose to use Wiener deconvolution due to its effectiveness and simplicity. We note that even though we design to be wavelength-invariant, it still has some residual wavelength dependence. The EDOF property that we engineer ensures that this variance is sufficiently small across the design bandwidth. Given the three primary colors in a typical camera sensor, we sample at three wavelengths using separate sources at 625 nm, 530 nm, and 455 nm. We simulate the images by convolving the experimental PSFs with the ground truths and adding Gaussian white noise (Fig.
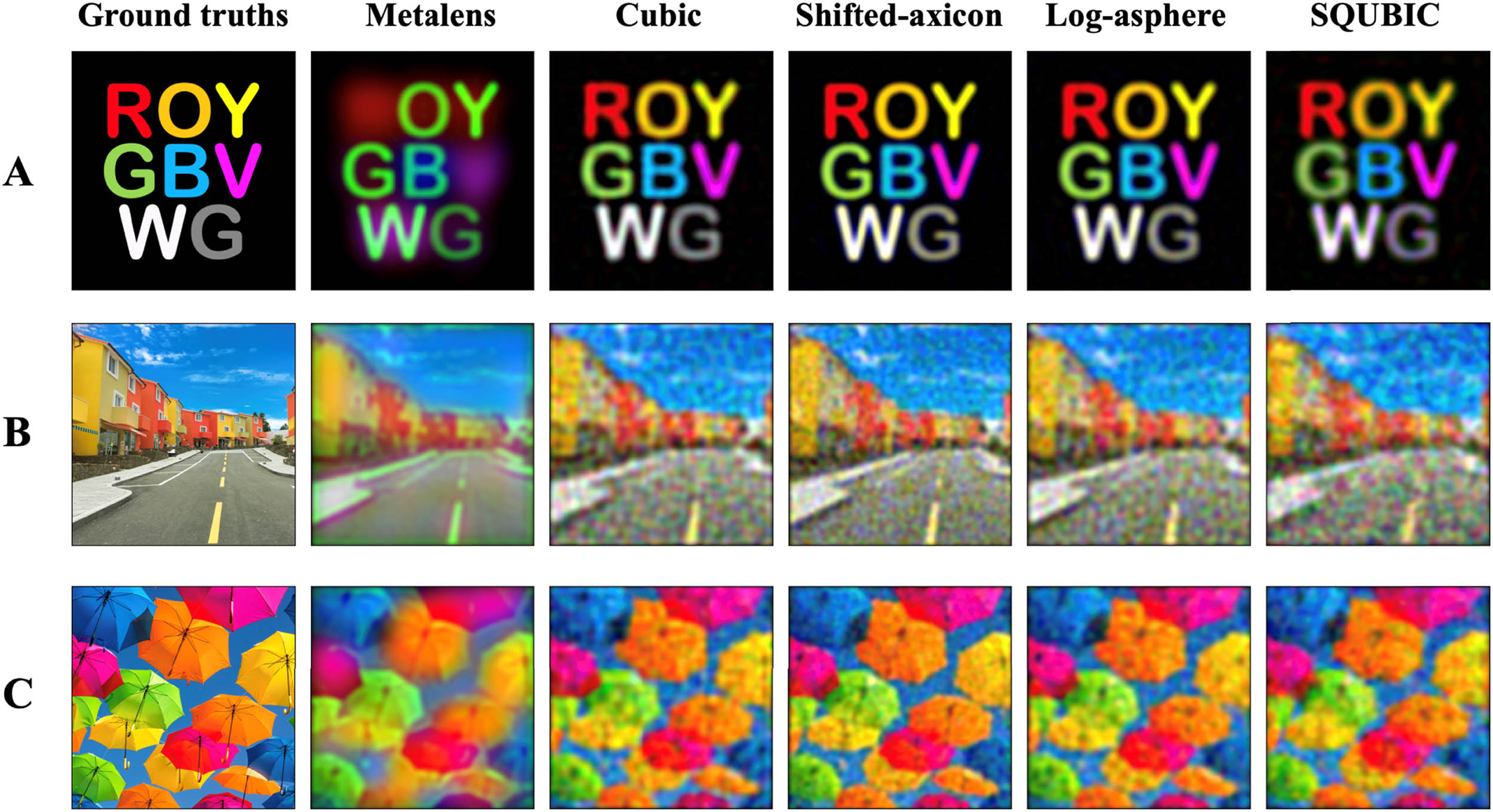
Fig. 3. Simulated imaging performance after deconvolution. Deconvolved images captured by the EDOF imaging system, using the simulated images and PSFs. The experimental counterpart can be found in Fig. 4 .
We then captured colored images projected by a SmallHD 5.5 in. Focus OLED HDMI Monitor using all the metasurfaces. We first record the spectra of the OLED monitor as well as the fiber-coupled LEDs. The individual spectra are recorded when displaying a single color at a time using a spectrograph (IsoPlane SCT320). The OLED monitor is placed away from the metasurface, which displays ground-truth images shown in Fig.

Fig. 4. Imaging performance. Restored images taken from (A) an OLED display of colored letters in ROYGBVWG, (B) a colorful neighborhood, and (C) vibrant umbrellas against the sky. The scale bar signifies 20 μm. Note that the metalens images are raw and unrestored.
We then characterized the experimentally captured images by calculating the SSIM for the “ROYGBVWG” image shown in Fig.

Fig. 5. Full color SSIM. The restored captures are scaled, rotated, and translated to align with the ground truth; then SSIM is calculated for each color channel for the metasurface.
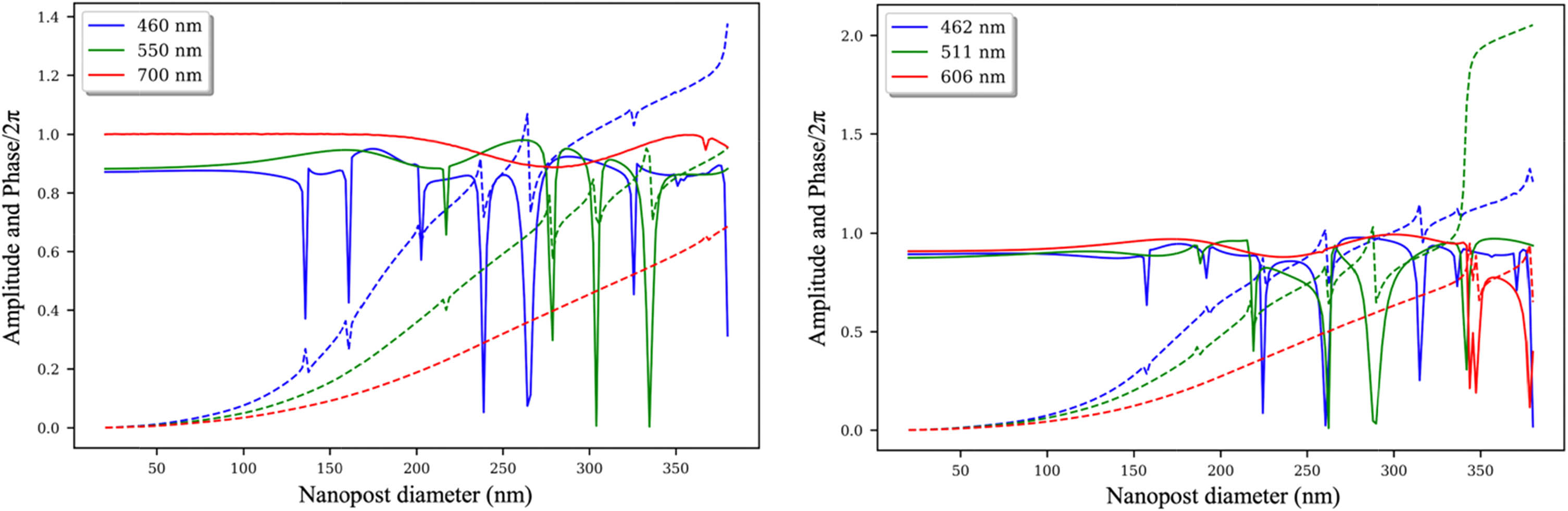
Fig. 6. Phase (dashed lines) and amplitude (solid lines) response of the nanopillars, simulated using RCWA.
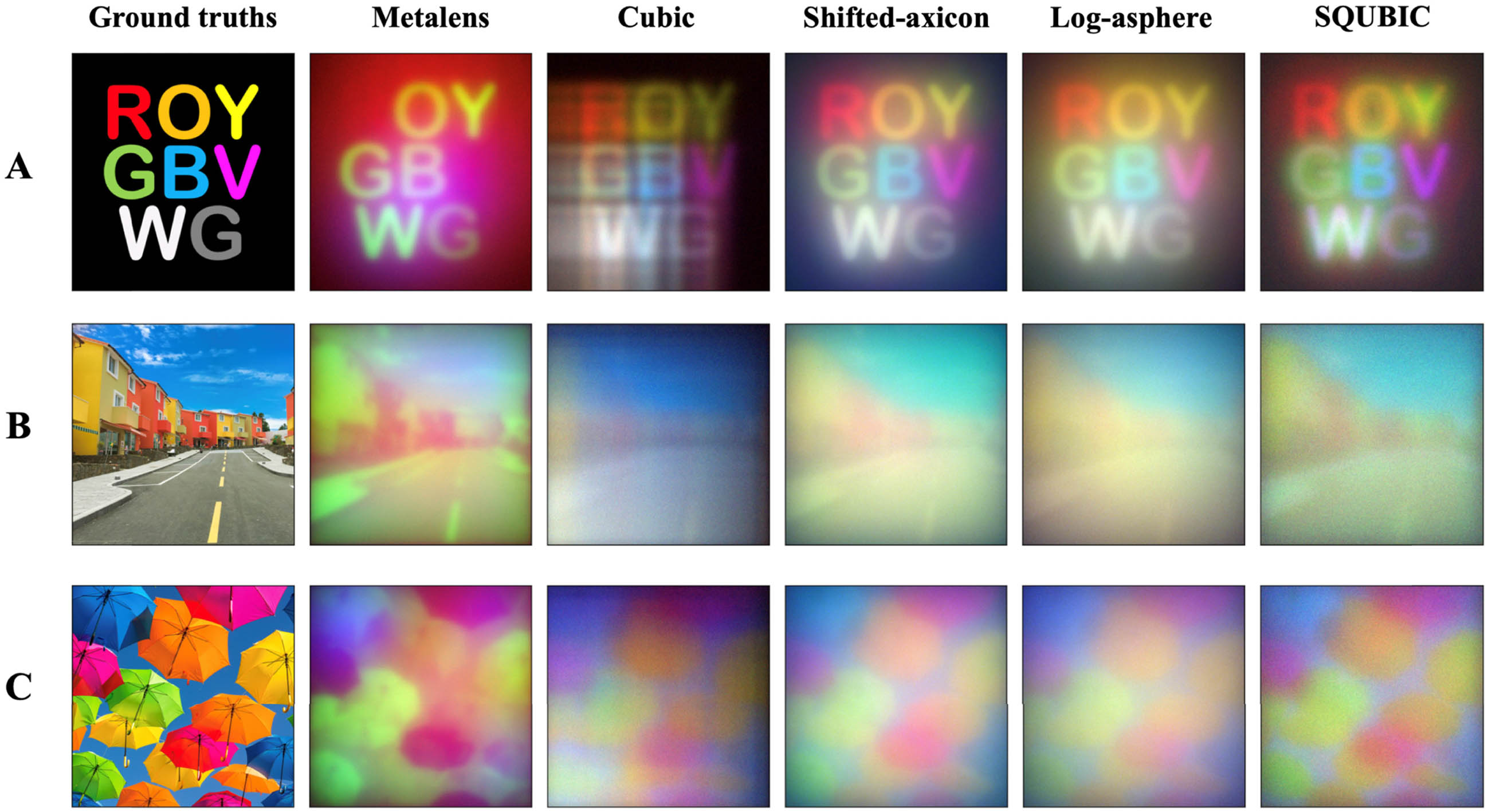
Fig. 7. Simulated captured images before deconvolution. The experimental counterpart is shown in Fig. 9 .
4. DISCUSSION
The SSIM results agree with the poor imaging quality exhibited by the metalens in the visible regime, caused by its strong chromatic aberrations. In contrast, the EDOF metasurfaces demonstrate an impressive ability to maintain a highly invariant PSF across a large spectral range. The imaging results as well as the SSIM calculations indicate that EDOF metasurfaces significantly outperform the standard metalens in full-color imaging. The log-asphere and shifted axicon designs both demonstrate the highest optical bandwidth for imaging. We note that recently a fundamental limit on the achievable optical bandwidth is reported given a thickness and numerical aperture of the lens [57]. For our parameters, the fundamental limit is , which is smaller than all the bandwidths demonstrated using EDOF lenses. While it is indeed possible to increase the optical bandwidth at the expense of image quality, it is difficult to estimate the Strehl ratio [58] for our EDOF lenses as the PSFs are very different from a lens and the final image is obtained only after deconvolution. Although previous works have demonstrated achromatic imaging with dispersion-engineered metalenses, our imaging system does not rely on any input polarization state and is generalizable to larger-aperture applications. A larger aperture will enable higher signal-to-noise ratio and faster shutter speed, which are crucial for practical applications. Moreover, dispersion-engineered metalenses require high aspect ratio scatterers and also multiple scatterers per unit cell, making the fabrication very challenging. In our approach the aspect ratios of the scatterers are relatively small, making the fabrication constraints more relaxed. Our image restoration process takes to restore a single image (pixel dimension: ; bit depth: 12 per color channel) on a single CPU. This process can, however, be significantly accelerated by utilizing multiple CPUs, graphics processing units (GPUs),or a dedicated field-programmable gate array. Preloading the MTF into memory will also speed up the computational reconstruction process. While this work uses Wiener deconvolution due to its speed, sophisticated deconvolution algorithms can be used to improve the image quality at the expense of time [59,60].
It is also important to emphasize the utility of rotational symmetric EDOF metasurfaces. A CPM is asymmetric and creates an accelerating Airy beam, which causes the PSF to shift for different wavelengths. This can be seen in the cross section of the 3D PSF in Fig.
5. SUMMARY
In summary, our imaging platform combines the form factor of ultrathin metasurfaces and the flexibility of computational imaging, making this an attractive solution for novel imaging applications. The CMOS compatibility of our silicon nitride platform, combined with a high NA of , makes this approach ideal for miniaturized microscopy, smartphone cameras, and endoscopy. Moreover, it is possible to increase the aperture of our EDOF hybrid system while maintaining the same NA and imaging characteristics. This aperture scalability combined with extremely lightweight and small size may open avenues to applications such as planar cameras as well as satellite imaging.
6 Acknowledgment
Acknowledgment. Part of this work was conducted at the Washington Nanofabrication Facility/Molecular Analysis Facility, a National Nanotechnology Coordinated Infrastructure (NNCI) site at the University of Washington, which is supported in part by funds from the National Science Foundation (NNCI-1542101, 1337840, and 0335765), the National Institutes of Health, the Molecular Engineering & Sciences Institute, the Clean Energy Institute, the Washington Research Foundation, the M. J. Murdock Charitable Trust, Altatech, ClassOne Technology, GCE Market, Google, and SPTS.
Luocheng Huang, James Whitehead, Shane Colburn, Arka Majumdar. Design and analysis of extended depth of focus metalenses for achromatic computational imaging[J]. Photonics Research, 2020, 8(10): 10001613.