Design of a barcode-like waveguide nanostructurefor efficient chip–fiber coupling
Download: 1128次
1. INTRODUCTION
A grating coupler is an enabling technique for the input–output (I/O) of chip scale interconnection [1–3]. Because of distinct advantages over other material systems, silicon photonics is a proven platform for optical interconnects. However, because the large refractive index contrast of optical waveguides fabricated on silicon-on-insulator (SOI), a huge mode field size mismatch exists between the fiber and the silicon submicrometer waveguide. Butt coupling with an inversed taper [4,5] or a grating taper [6] could be used to interface the optical fiber and the submicrometer sized waveguide, but this requires facet polishing and is not compatible with wafer scope testing. A grating coupler is another option that has been shown to be an efficient light coupling technique in silicon photonics [7–9].
Several practical issues arise before grating coupler can be implemented as the I/O solution. In particular, a grating coupler with vertical light diffraction is desired as it has smaller packaging difficulties compared with off-normal fiber coupling. However, this type of grating suffers from second-order Bragg reflection, which limits coupling efficiency. To overcome this limit, a slant grating structure [10], a chirped grating [11], and a quasi-periodic multiple slot grating [12] were used to suppress the backreflection. However, a periodic grating structure with one or two etching steps is normally designed based on first principles with limited degrees of freedom, and it consumes much operational expenses. In addition, the manual design becomes complicated if more etching steps are allowed due to fabrication advances. Hence, it would be beneficial if nanophotonic devices could be designed by algorithm. In [12], a genetic algorithm was used by Covey and Chen to demonstrate a grating coupler, but with a dual layer horizontal slot waveguide structure, which is not standard for photonic integrated circuits. Piggott
Another issue with silicon grating couplers is the polarization dependence. There has been much work reported to realize a polarization independent or insensitive grating coupler. Two-dimensional (2D) polarization diversity circuits were used, but this doubles the chip area [14,15]. Subwavelength structures balancing the effective index between TE and TM were also proposed to achieve polarization independence [16,17]. More recently, a tapered grating nanostructure was demonstrated to achieve polarization-insensitive coupling [6]. However, they all require an elaborate design process. Because of the large index difference for TE and TM, a polarization-insensitive grating coupler is much more difficult to design than is a single-polarization case. Thus. developing an automatic design approach becomes more important.
In this paper, we utilized a modified direct binary search algorithm to automatically design a barcode-like one-dimensional (1D) nanostructure for chip–fiber coupling. A QR-code-like 2D subwavelength nanostructure designed by algorithm has been demonstrated as an ultracompact polarization beam splitter [18], wavelength multiplexer [19], and for waveguide bending [20]. Here we show that a discretized waveguide nanostructure could be designed by algorithm for different functionalities. We obtain two structures, with one for chip surface normal coupling to a vertically located fiber and the other for polarization-independent coupling.
2. OPTIMIZATION PROCESS
A conventional periodic grating coupler is normally fabricated with no more than two etching steps. The designer must carefully optimize the fill factor and the grating period to minimize the backreflection and match the coupling strength with the fiber mode profile.
Here we introduce a modified direct search algorithm to automatically design a diffractive nanostructure for chip–fiber coupling. We consider an SOI substrate with 340 nm top silicon and 3 μm buried oxide (BOX). The coupling area was discretized equally into 125 pixel lines along the light propagation direction, and each line is a narrow slab with 120 nm width. The narrow silicon slab thickness is divided equally into four- and eight-level heights of the total 340 nm top silicon, similar to the digital multilevel modulation formats. Therefore, the effective index of each slit can be engineered with more flexibility by choosing different thicknesses. A side-view schematic of the proposed nanostructure is shown in Fig.
3. RESULTS AND DISCUSSION
3.1 A. Vertical Coupling Nanostructure Design
First we investigate the possibility of getting a surface normal coupling design using a computer-optimized barcode-like nanostructure. We first define a random nanostructure to initialize the design process. The optimization process iterates based on the modified direct search algorithm described above. The FOM we are interested in here is coupling efficiency, which is calculated by taking the overlap between the fiber mode field and the diffraction field into account. The convergence radius
On the other hand, the more levels we define, the more freedom we have to optimize the diffraction structure. From the standpoint of fabrication, it would be challenging to use more than two etching steps, but the focusing ion beam writer could be a promising option to fabricate such multilevel nanostructures [21].
We use a 4-core standard desktop computer operating at 4 GHz. The design process of the nanostructure with vertical coupling is completed within 72 h. The side view of the optimized nanostructure schematic is shown in Figs.
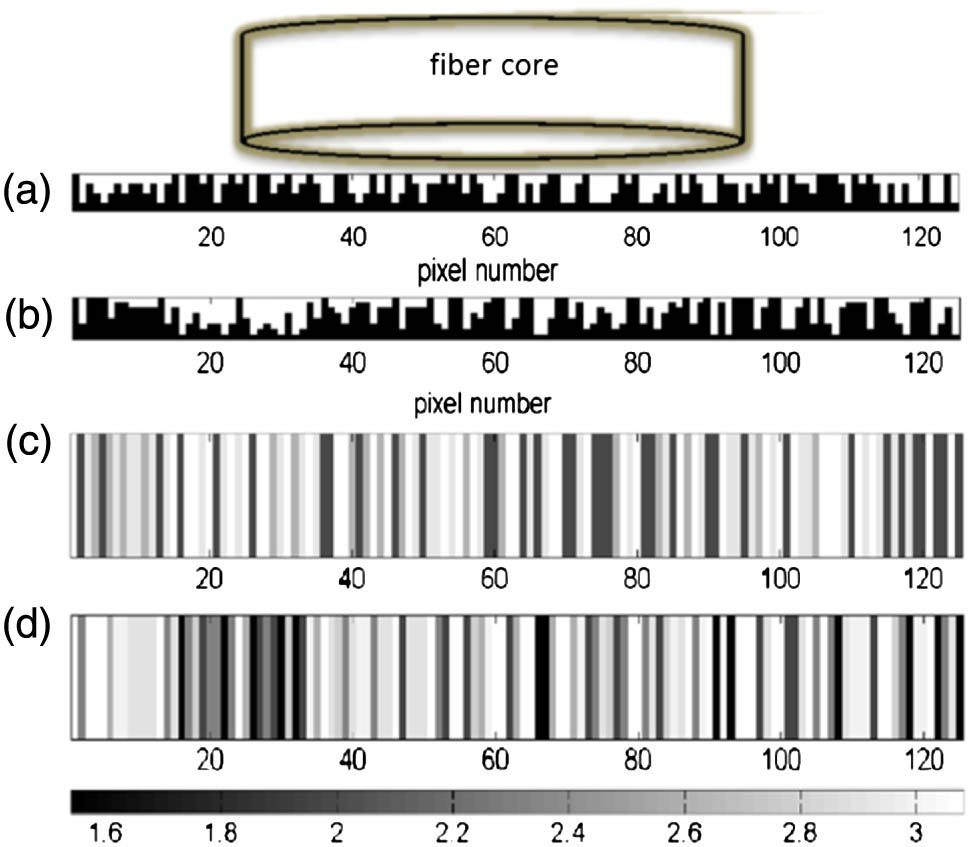
Fig. 2. Side-view schematics of the optimized coupling nanostructures for (a) four levels and (b) eight levels. Gray-scale representations of the discretized effective indices of the (c) four-level and (d) eight-level vertical coupling nanostructures.
During the FDTD simulation, we set a power monitor at the bottom of the BOX layer, and the obtained value indicates that the leakage into the substrate is quite small, as shown in Fig.

Fig. 3. (a) Simulated amount of light leakage to the substrate. (b) Backreflection of the designed nanostructure. (c) Coupling efficiency of the two types of nanostructure.
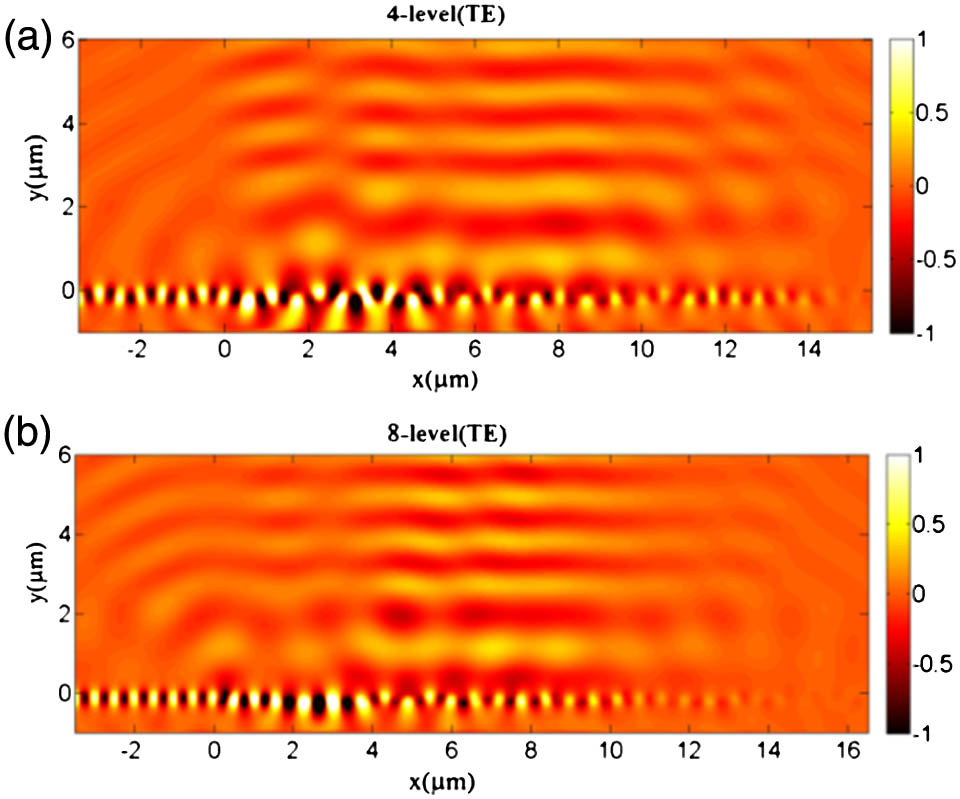
Fig. 4. Simulated optical field distribution of the diffracted wave for the (a) four-level and (b) eight-level nanostructures, respectively.
3.2 B. Polarization-Independent Nanostructure Design
In addition to the coupling angle, polarization dependence is another tough issue for a high index contrast grating coupler. Here we propose to optimize the diffractive nanostructure to achieve polarization independence. For a polarization-insensitive nanostructure, we have to define two output objectives, which are the coupling efficiencies for the quasi-TE and quasi-TM waveguide modes. Thus, it becomes a multiple objective problem, and the general convergence condition for such problem can be expressed as
The entire design consumes about 144 h using the same desktop as we described before. The optimized structure schematic for polarization-independent coupling is shown in Fig.

Fig. 5. (a) Side-view schematic of the optimized eight-level polarization-independent nanostructure. Gray-scale representations of the discretized effective indices of the optimized structure for (b) TM and (c) TE.
The light diffracted toward the substrate is monitored in the simulation and the results are plotted in Fig.
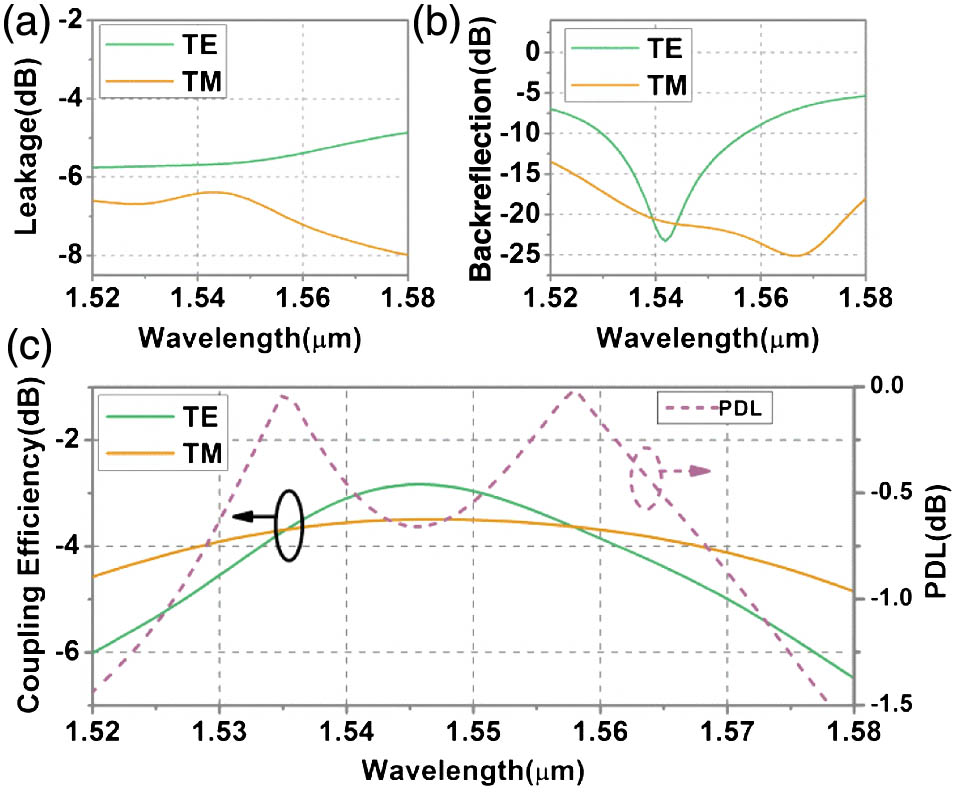
Fig. 6. (a) Simulated amount of light leakage to the substrate. (b) Backreflections of the TE and TM modes from structure to waveguide. (c) Coupling efficiencies of the TE- and TM-mode light coupling from waveguide to single-mode fiber in the polarization-independent nanostructure.
The simulated field distribution of light diffraction from the optimized eight-level grating coupler is shown in Fig.
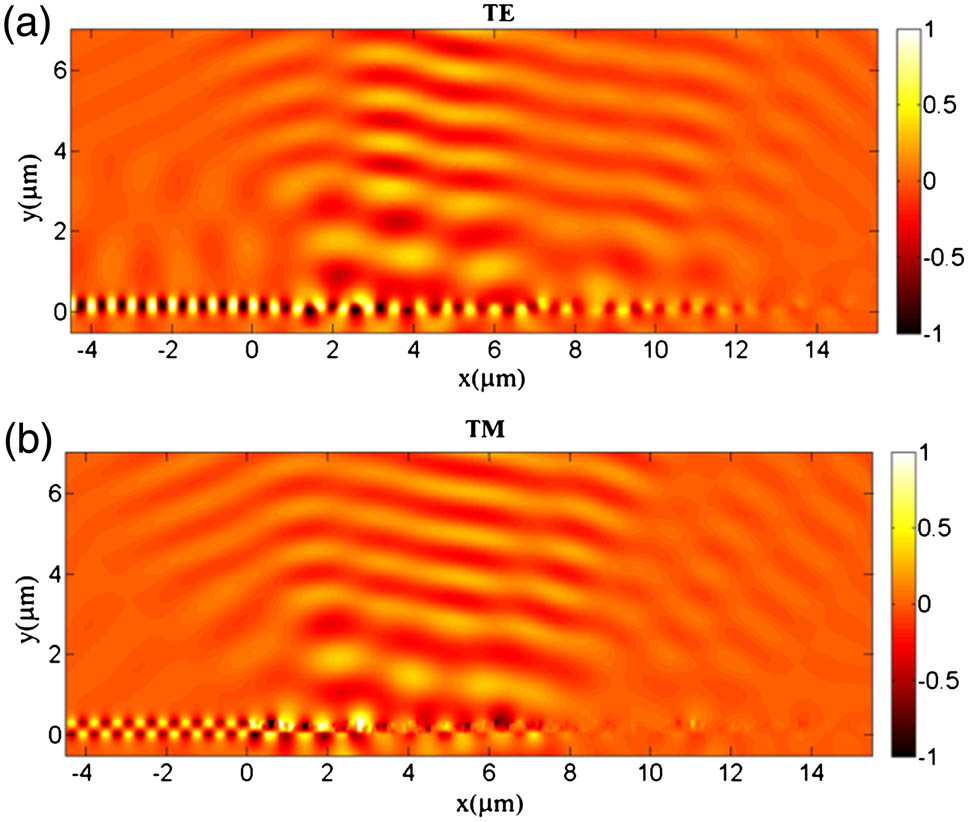
Fig. 7. Diffractive electric field of the eight-level structure for (a) TE and (b) TM polarization.
4. CONCLUSION
We have proposed a barcode-like 1D nanostructure for chip–fiber coupling using a modified direct search algorithm. We have addressed the coupling angle and the polarization issue, both of which are difficult to address in a periodic grating. The algorithm can be regarded as a general method to design a coupling nanostructure with multiple objectives, such as bandwidth, coupling efficiency, polarization, and coupling angle. A nanostructure with surface normal diffraction is achieved, and the efficiency is as high as
Article Outline
Xiang Wen, Ke Xu, Qinghai Song. Design of a barcode-like waveguide nanostructurefor efficient chip–fiber coupling[J]. Photonics Research, 2016, 4(6): 06000209.