Non-resonant magneto-optical effects in cold atoms
Download: 995次
The development of atomic clocks has led to many scientific and technological advances such as the Global Positioning System (GPS)[1], the Internet (which depends critically on frequency and time standards), high-resolution spectroscopy[2], and so on. The most accurate atomic clock uses cold atoms with a temperature close to absolute zero as the medium. Generally, the clock signals are detected by using the absorption method which produces additional background noise, thereby reducing the signal contrast and signal-to-noise ratio (SNR)[3]. In a rubidium atomic clock experimental setup[4], Faraday rotation is used to detect the clock signal in a vapor cell to improve its contrast[5,6]. As for cold atom clocks, several methods for laser cooling of atoms have been reported. Integrating sphere cooling has a simpler structure, occupies a smaller volume, and does not require precise collimation in the cooling light[7]. These advantages render integrating sphere cooling an ideal cold atom source for a compact and small cold atom clock. We have observed the Faraday rotation signal in cold rubidium atoms in an integrating sphere which addressed the previously mentioned problem by removing the additional background noise[8].
In this Letter, we present a different detection method with another probe light level, which brings out several desirable experimental results for rotational signals. In our previous scheme[8], cold rubidium atoms were first obtained by a cooling laser (Toptica TA100) locked to the transition of
Magneto-optical effects arise when light interacts with a medium in the presence of a magnetic field
Equations (
The experimental setup is shown in Fig.
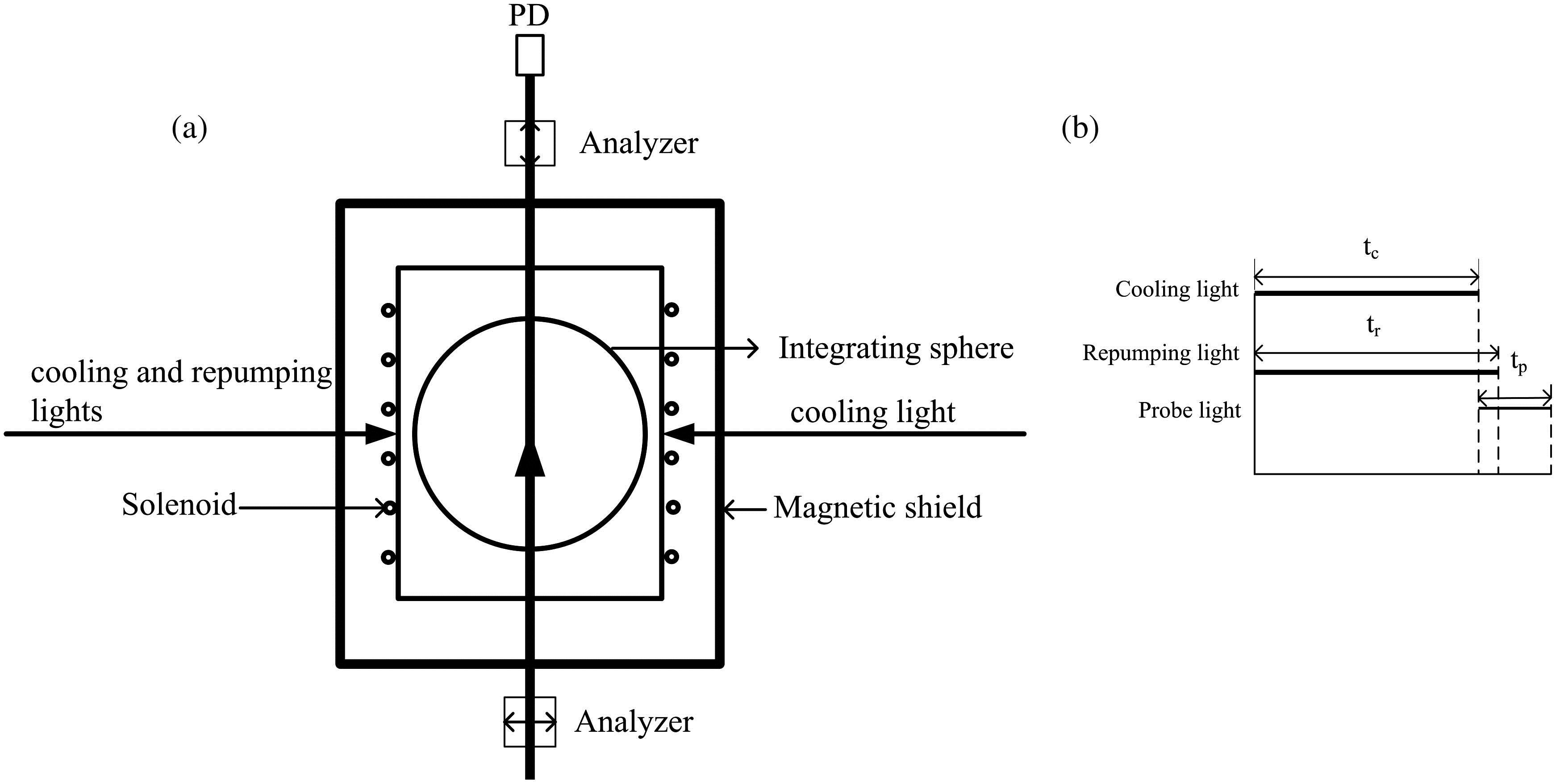
Fig. 1. (a) Experimental setup for magneto-optical detection; (b) timing sequence of the experiment. Cooling time and the repumping time are 177.5 and 179 ms, respectively. Probe time is 7.5 ms which is switched on immediately after the cooling light is turned off.
The power of the two cooling lights which travel along the opposite directions is 130 mW, and the power of the repumping light is 5.5 mW. We can explore the rotation angle versus the magnetic field according to Eqs. (

Fig. 2. Transmitted probe light intensity when the biased magnetic field is 20.6 and 0 mG (solid and dashed lines, respectively). There is repumping light leakage at ; pure transmitted probe light intensity is obtained by subtracting such repumping light leakage.
The transmitted rotation angles of the linear probe light versus the magnetic fields at different intensities of the probe light are shown in Fig.
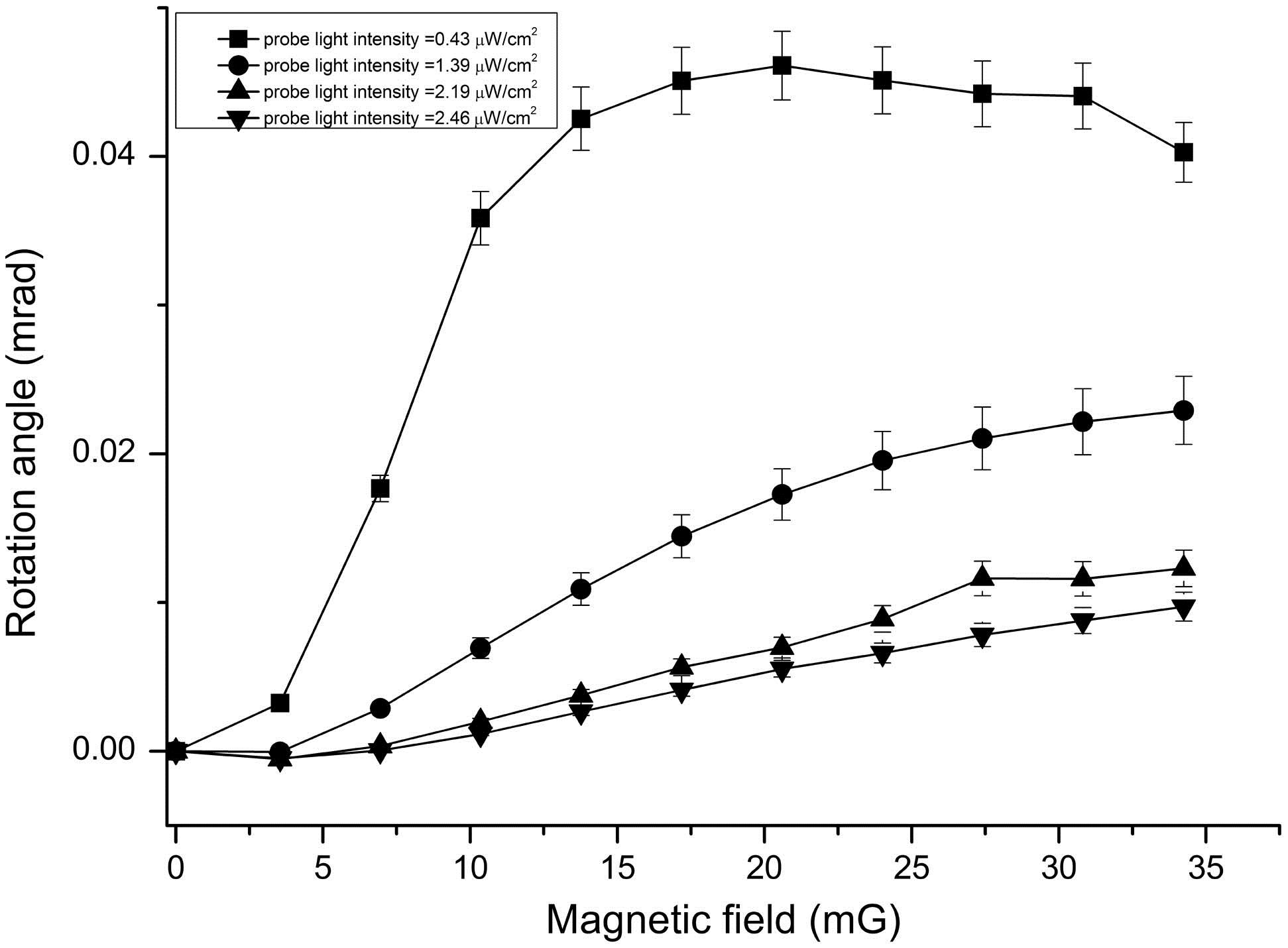
Fig. 3. Rotation angle versus the biased magnetic fields at different incident probe powers where the uncertainty of the data points is 5%. Probe light transition is from to . Magnetic field is from 0 to 34.2 mG. Incident probe intensities are 0.43, 1.39, 2.19, and . Largest rotation angle is 0.046 mrad when the magnetic field is 20.6 mG and the probe light intensity is .
The magnetic field was set as 20.6 mG to investigate the rotation angle versus the probe light intensity (Fig.

Fig. 4. Rotation angle versus the probe light intensity where the uncertainty of the data points is 5% at different detunings 0, , and, −6.3 MHz, respectively. Probe light transition is from to , and the magnetic field is set as 20.6 mG. Profiles are similar as for different probe detunings; there is a peak at about and then decreases slowly.
We then set the magnetic field at 20.6 mG and the incident power at
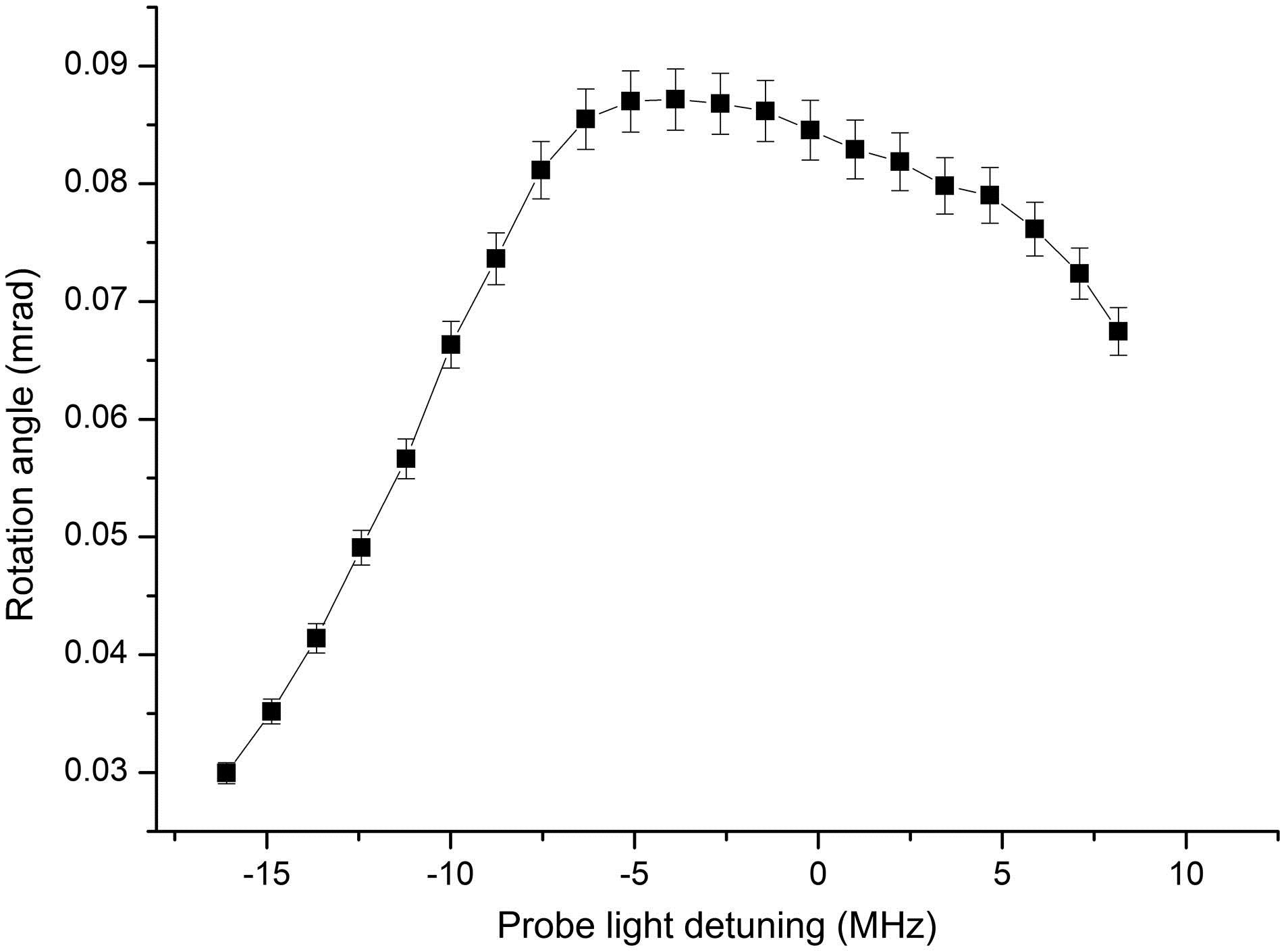
Fig. 5. Rotation angle versus probe light detuning where the uncertainty of the data points is 5%. Probe light transition is from to . Magnetic field is 20.6 mG and the incident power is . Maximum rotation angle of 0.087 mrad is at red detuning .
In conclusion, we present nonresonant magneto-optical effects in cold rubidium atoms in an integrating sphere. The rotation angle of the linear polarized probe light at different magnetic fields, the incident probe power, and the probe detuning are demonstrated. It is found that the rotation angle profiles versus incident probe power are similar for different probe detunings, and the value is nearly fixed from a red detuning 5 MHz to the resonant frequency. The probe scheme of
[1]
[2]
[3]
[4]
[5]
[6]
[7]
[8]
[9]
[10]
[11]
[12]
Jinyin Wan, Huadong Cheng, Yanling Meng, Ling Xiao, Peng Liu, Xiumei Wang, Yaning Wang, Liang Liu. Non-resonant magneto-optical effects in cold atoms[J]. Chinese Optics Letters, 2015, 13(2): 020201.