Dynamically pre-compressed hydrocarbons studied by self-impedance mismatch
1 I. INTRODUCTION
The behavior of hydrocarbons under extreme conditions is of great interest across the field of high-energy-density science. Consisting of two of the most common elements within giant planets, they serve as an excellent example for study of the behavior of planetary interiors.
In an experiment, off-Hugoniot states will tend to have a lower temperature than if the same pressure were reached with single shock. This is easily shown for a polytropic gas, where the entropy increases as a function of the pressure ratio P1/P0 across the shock such that multiple weaker compressions give a smaller rise in entropy,
The principal difficulty in studying off-Hugoniot states experimentally is the lack of previous experimental data. While there exists (e.g., in the case of plastics) a large body of work from single-shock compressed samples,
This paper presents measured results for the pressure, density, and reflectivity of polystyrene
2 II. EXPERIMENT
The experiment was carried out using the Shenguang-III prototype laser, located at the Research Center of Laser Fusion (LFRC) at the China Academy of Engineering Physics (CAEP) in Mianyang, China.
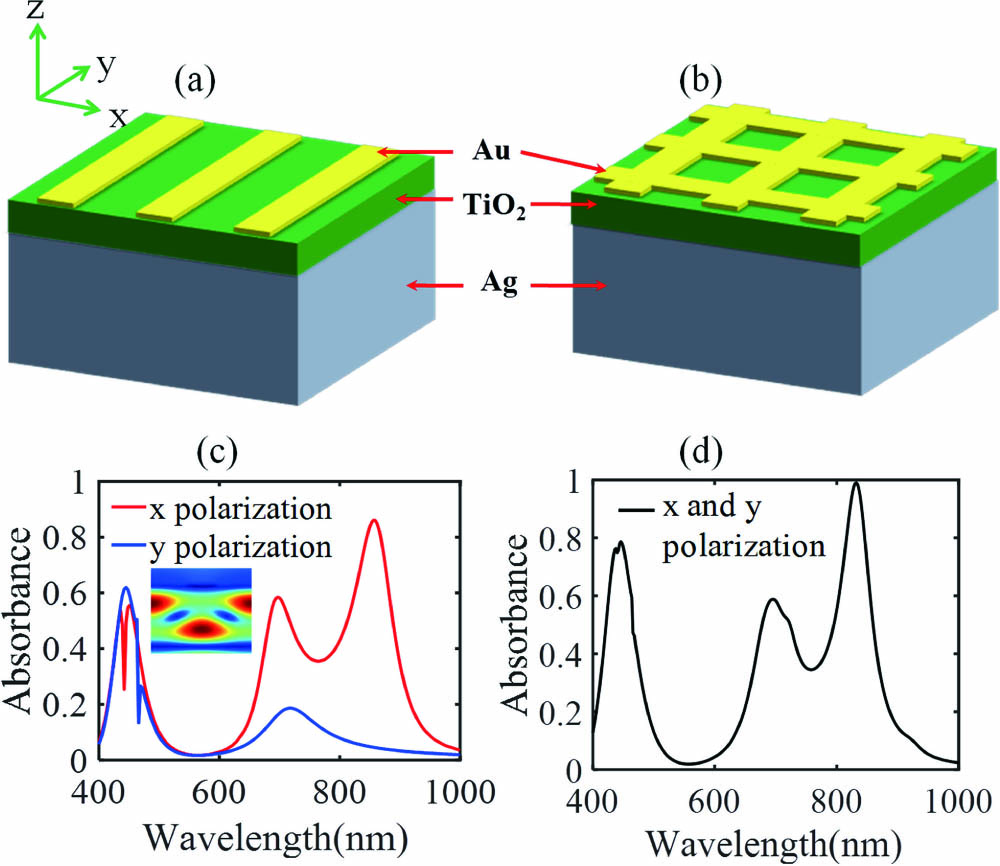
Fig. 1. Cartoon of the experimental setup, showing the hohlraum and the target package, consisting of Al–Au–Al layers attached to the CH sample. The driving radiation temperature is monitored on the XRD cameras, and shock propagation data are taken on the VISAR system.
The soft X-ray spectrum generated in the hohlraum was monitored by X-ray diode (XRD) detectors; examples of the temporal evolution of the temperature for the single- and double-shock cases are shown in
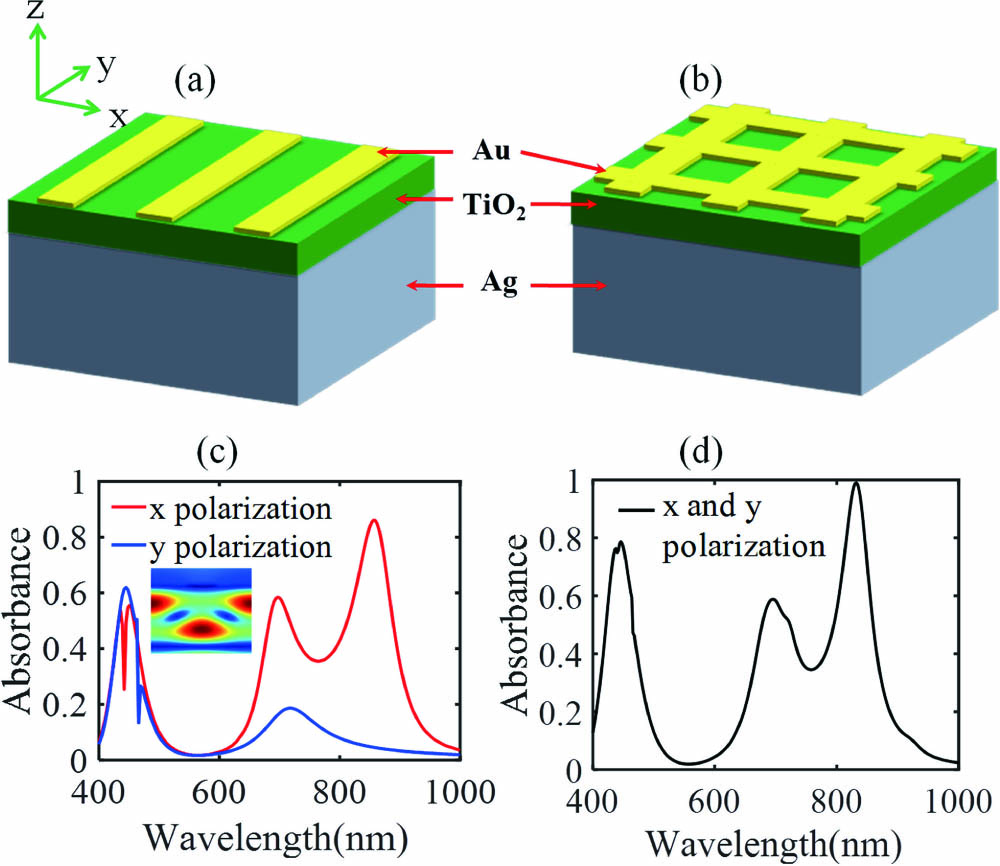
Fig. 2. Examples of the measured radiation temperature incident on the ablator surface as a function of time for the cases of a single and a double shock. The shaded uncertainty is the variation in measured temperature between the cameras. The lineouts correspond to the temperatures in shots 302 and 315, respectively (see Table I in the Appendix ).
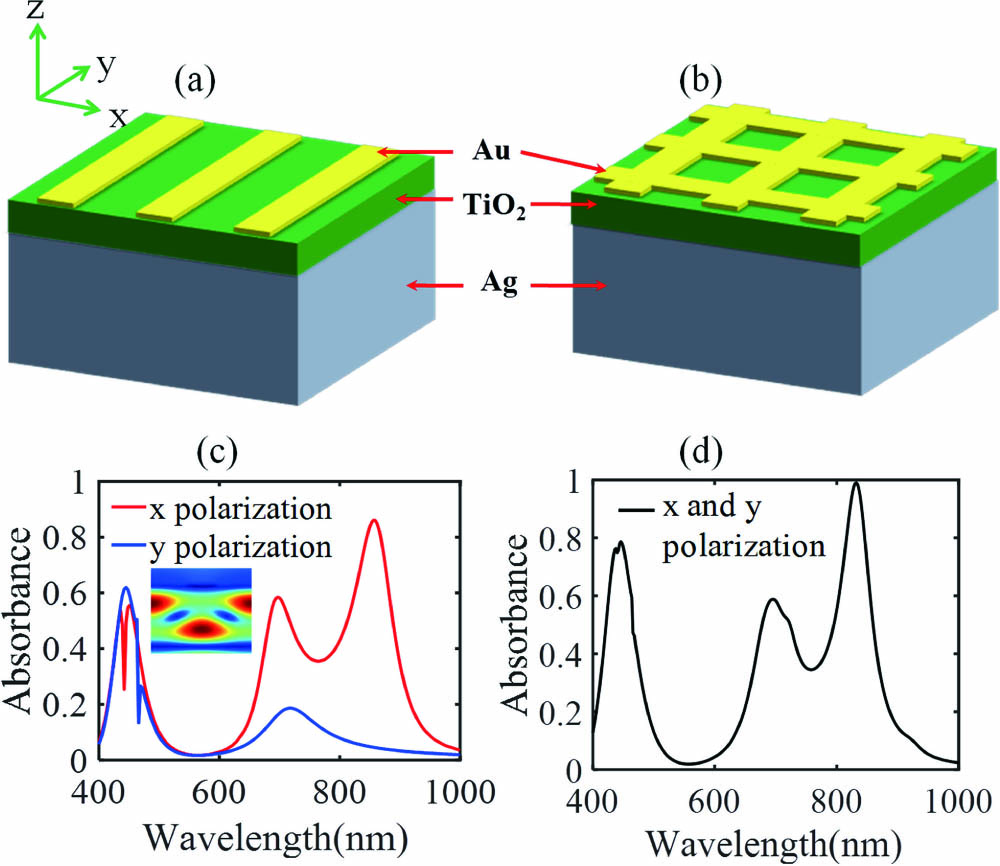
Fig. 3. Plastic target density, simulated using the double-shock radiation profile in the radiation hydrodynamics code HELIOS, with SESAME equation of state 7592 for polystyrene. The radiation is incident from the left-hand edge onto the ablator; the shock passes through this and into the CH, the boundaries of which are marked by the black lines. The coordinates are Eulerian, allowing the different shock-front velocities to be observed.
The behavior of the sample was monitored using two velocity interferometer system for any reflector (VISAR) arms, at a probe wavelength of 532 nm. The velocity per fringe (VPF) parameters of the left and right arms were 7.116 km/s and 4.981 km/s, respectively. The time windows for the VISAR data acquisition were generally around 10 ns (9.76 ns on the left arm and 10.27 ns on the right arm), although some shots used a 5.45 ns time window on the right arm to give finer time resolution.
3 III. ANALYSIS
3.1 A. Self-impedance mismatch technique
The self-impedance mismatch technique determines the state of a double-shocked material from the initial and final conditions, both of which lie on the Hugoniot, and the speed of the second shock. A full description of the method can be found in the paper by Guarguaglini et al.,
Considering the example shown in
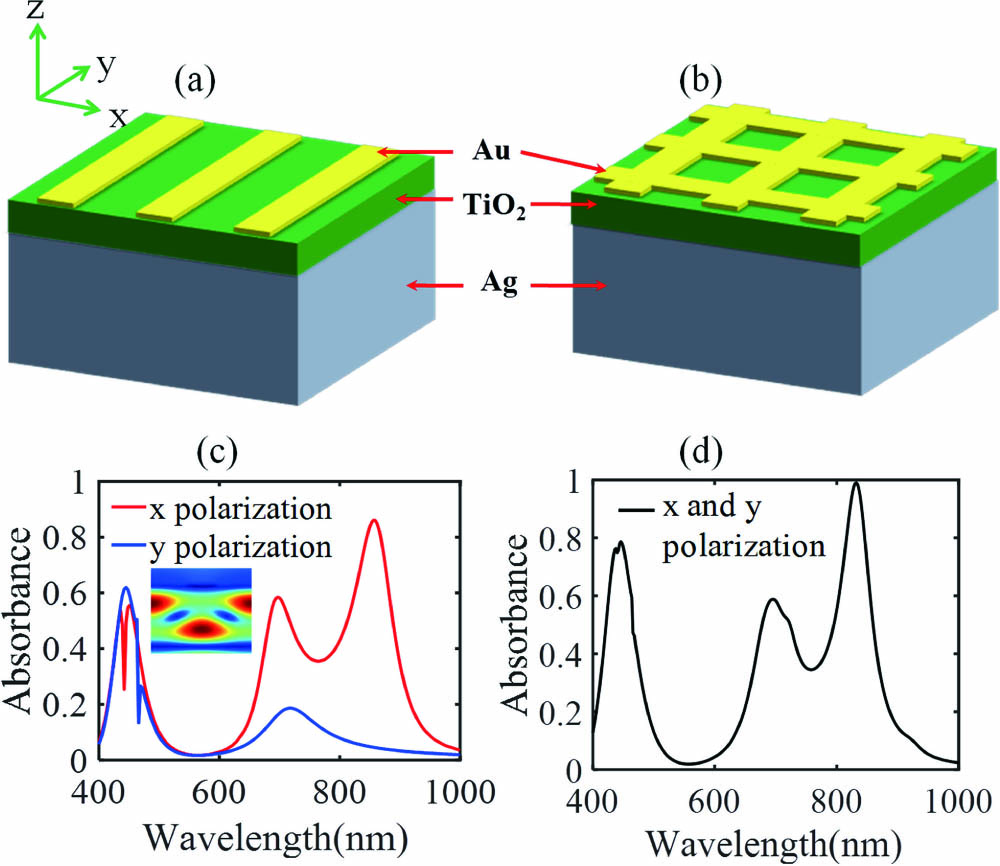
Fig. 4. VISAR data, calculated velocities, and corresponding reflectivity values. Between t 1 and t 2, the value is equal to the apparent particle velocity u app, while the higher velocity from t 3 to t 4, seen only on one of the VISAR arms, is due to the merged shock u s ,m . The reflectivity values show a rise after the first shock enters the plastic, possibly due to changes in the Al–CH interface, with the horizontal dashed line showing the theoretical Al–plastic reflectivity of 88%. The flat region of nonzero reflectivity between t 2 and t 3 provides the reflectivity value for the double-shocked state. The data are from shot 315 (see Table I in the Appendix ).
3.2 B. Application and results
An example of the VISAR data is shown in
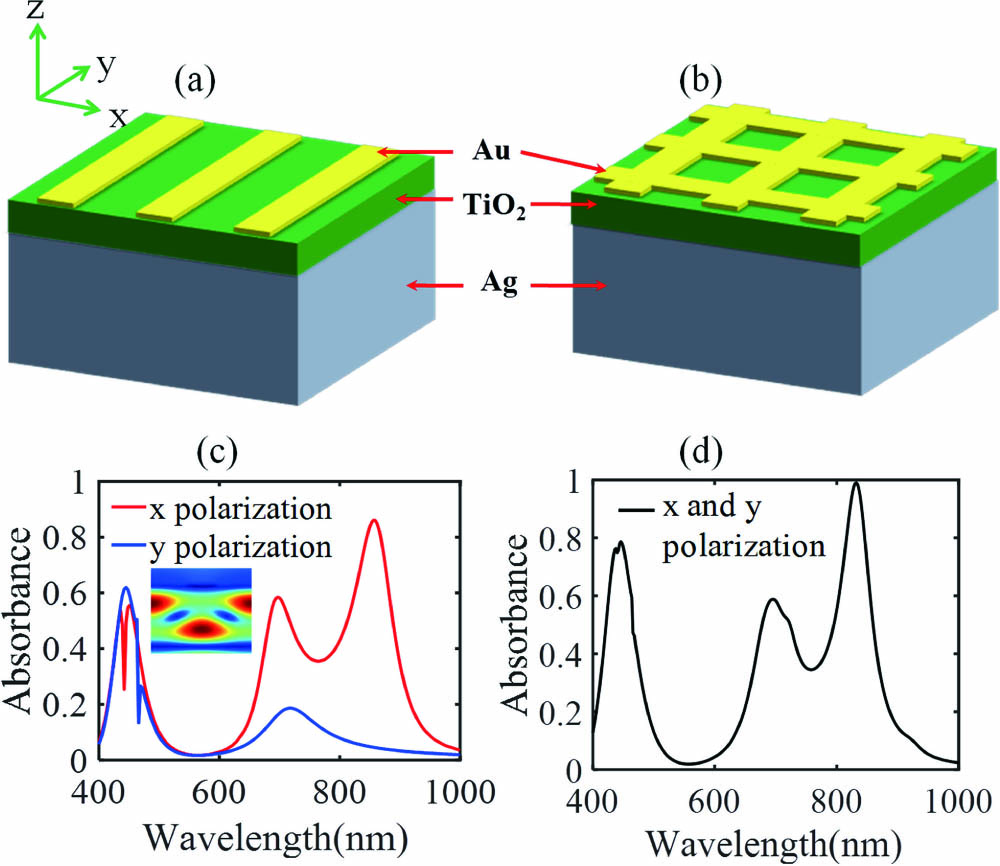
Fig. 5. Example of the self-impedance mismatch technique illustrated on the P -u p plane. The double-shocked state P2 is determined by the intersection of the Rayleigh line from the single-shocked state P1 and the release line of the final state P3. The uncertainty in the Rayleigh line, indicated by the shaded orange area, is due to the uncertainty in the transit time, and hence the shock speed u s ,2, while the shaded gray area is due to uncertainty in the merged shock velocity u s ,m , and hence the final state. The uncertainties in pressure and density are taken from the intersection of the shaded areas, and are particularly large for the density ρ .
In the case of polystyrene, the change in the index of refraction after shock compression has been well studied; using the empirical relations for n(P) from Zhang et al.
For the final state reached by the single merged shock, after time t3, the VISAR laser reflects from the shock front, and so the apparent velocity is related to the shock velocity by
The last quantity to be extracted from the VISAR data is the speed of the second shock us,2, which is necessary to define the slope of the Rayleigh line. Under the conditions reached in this experiment, this shock is only very weakly reflecting, and so the velocity cannot be calculated from fringe shifts, but must instead be deduced from transit time measurements and the initial shock and particle speeds. In the case considered, we find a transit time Δt = t3 − t2 = 0.25 ± 0.04 ns, for a shock velocity
Substituting the measured velocities into Eqs.
While this does demonstrate the capability of the self-impedance mismatch technique to directly determine off-Hugoniot conditions, the results, particularly for the density, show very large error bars. Since this is due mainly to the uncertainty in the second shock speed us,2, future experiments could consider approaches to determine this more precisely. While the best way to do this would be driving a shock that was strong enough to be reflecting, so that it could be measured directly, the interface with the pre-compressed material means that it would probably need to be so strong that the benefits of the double-shock drive would be lost, since we would be beyond conditions relevant for planetary interiors. Instead, a thicker plastic sample, and a longer delay between the shocks entering it, should increase the time t2 − t3, such that an uncertainty of the order of ±0.04 ns has a smaller overall effect.
Although we were not able to measure the temperatures directly in this experiment, we have estimated them from known equation-of-state (EOS) data, using SESAME equation of state 7592 for polystyrene. The temperatures were estimated from the measured (P, ρ) position, with the uncertainty in the temperature arising solely from uncertainties in these quantities. This is slightly less than would be expected if the temperature were measured directly, such as by streaked optical pyrometry,
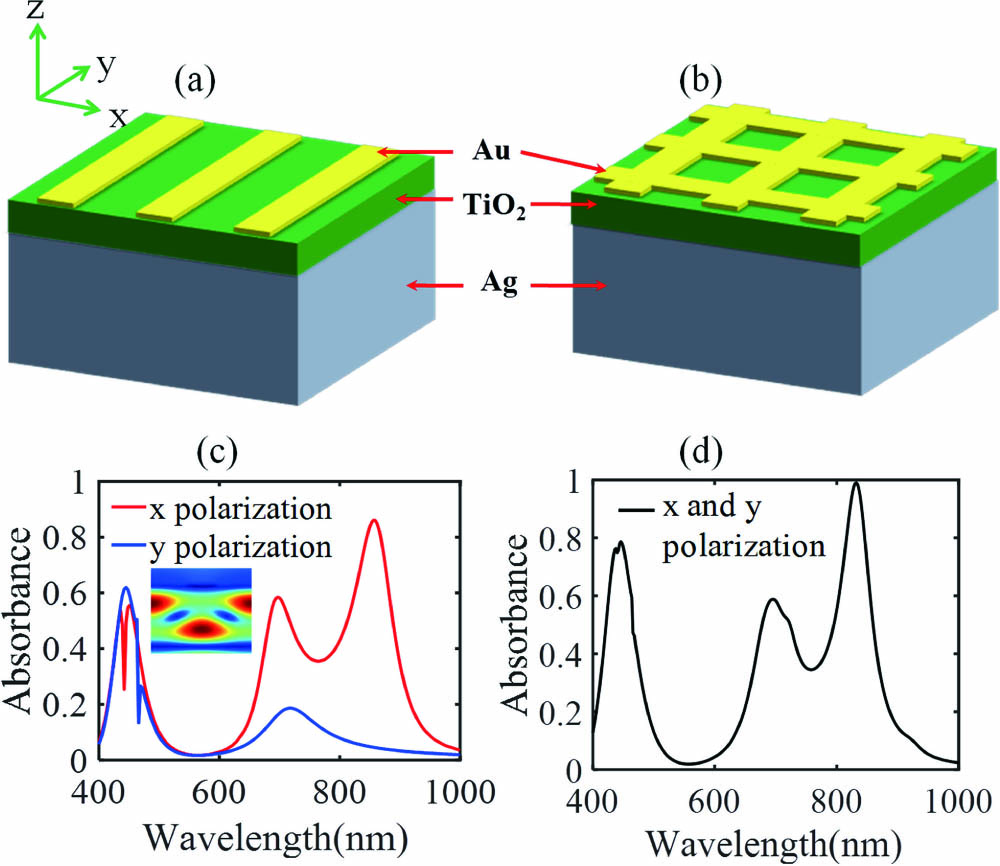
Fig. 6. Pressure–temperature phase diagram, showing the conditions reached on the Hugoniot (red triangles and dashed line), and with the double-shock drive (green squares and dot-dashed line); the highest-pressure point on the Hugoniot is omitted so that the lower-temperature results can be distinguished. Also shown are the conditions where diamond formation from double-shocked CH was observed by Kraus et al. 1 (blue diamonds), the expected melting line of diamond from Wang et al. 20 (blue dashed line), and the hydrocarbon demixing boundary from Gao et al. 21 (black dotted line).
3.3 C. Reflectivity
As well as the fringe shift, the VISAR data can be used to extract values for the reflectivity of the sample, in both the single- and double-shocked states. The reflectivity values are calculated by first subtracting the average value at late times (t > 3.5 ns for the example in
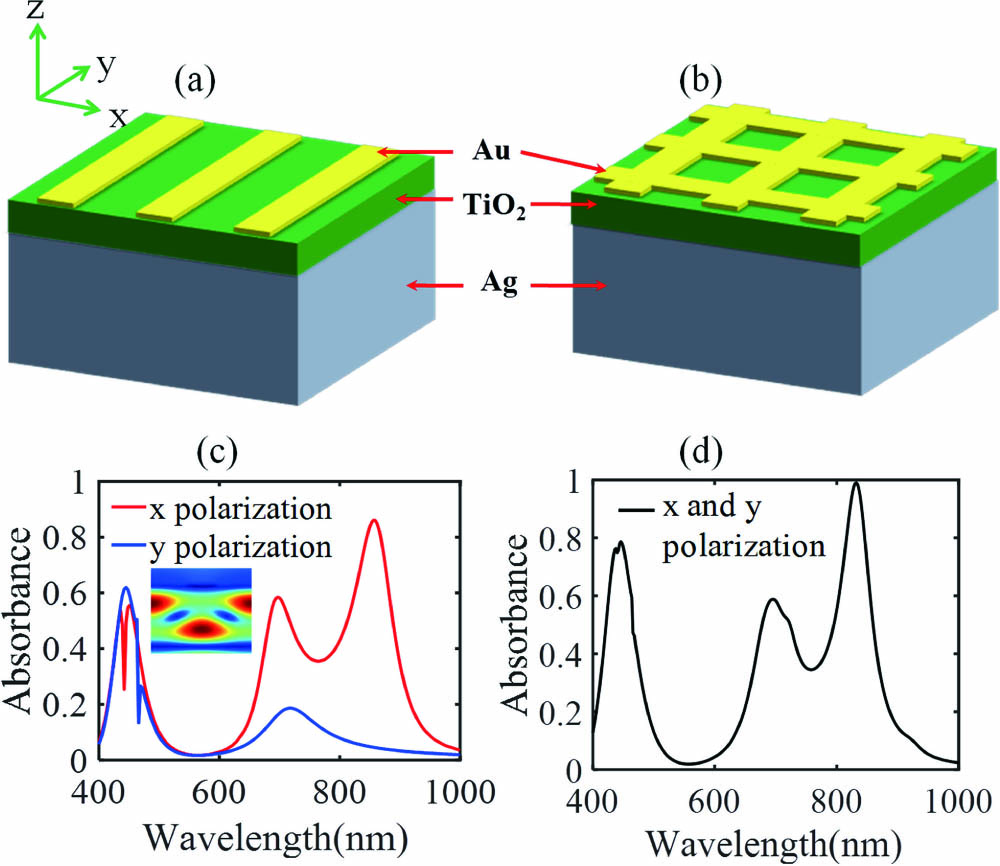
Fig. 7. Reflectivity of shocked polystyrene as a function of (a) pressure, (b) density, and (c) temperature, all taken with probing wavelength λ = 532 nm. The red triangles show points on the Hugoniot, either from single shocks or from the final-state merged shocks, while the green squares show double-shocked conditions. The closed and open green squares show reflectivity values before and after correction, respectively, as described in the text. The blue crosses are results from the OMEGA facility, taken from Barrios et al. 11 The disagreement at high pressures is likely due to a systematic offset in the initial reflectivity, as this was not measured prior to the shot.
These measured reflectivity values can be compared with previous data from highly compressed hydrocarbon samples, taken by Barrios et al.
While the normalization issue is less of an issue for the double-shock shots, comparing the reflectivity results with previous work is more challenging. Since they are not expected to be transparent, the refractive index n2 must be replaced with its complex value
To compare the double-shocked states with states on the Hugoniot, we would like to “correct” the measured reflectivity to give the value that would be expected if it were adjacent to the uncompressed, rather than pre-compressed, CH, i.e., evaluating Eq.
The value n2,r can be used along with the ambient CH n0 to give a “corrected” reflectivity from the double-shocked state. Alternatively, any of the (n2, κ) pairs that reproduced the observed reflectivity can be used. It is empirically seen that the maximum corrected value for the reflectivity is that calculated with n2 = n2,r and κ = 0. Therefore, the corrected reflectivity that can be obtained under this simplifying assumption is the maximum possible value that the reflectivity could take, and accounting for the extinction coefficient in the refractive index, κ, would reduce the corrected value. These maximum corrected values are plotted in
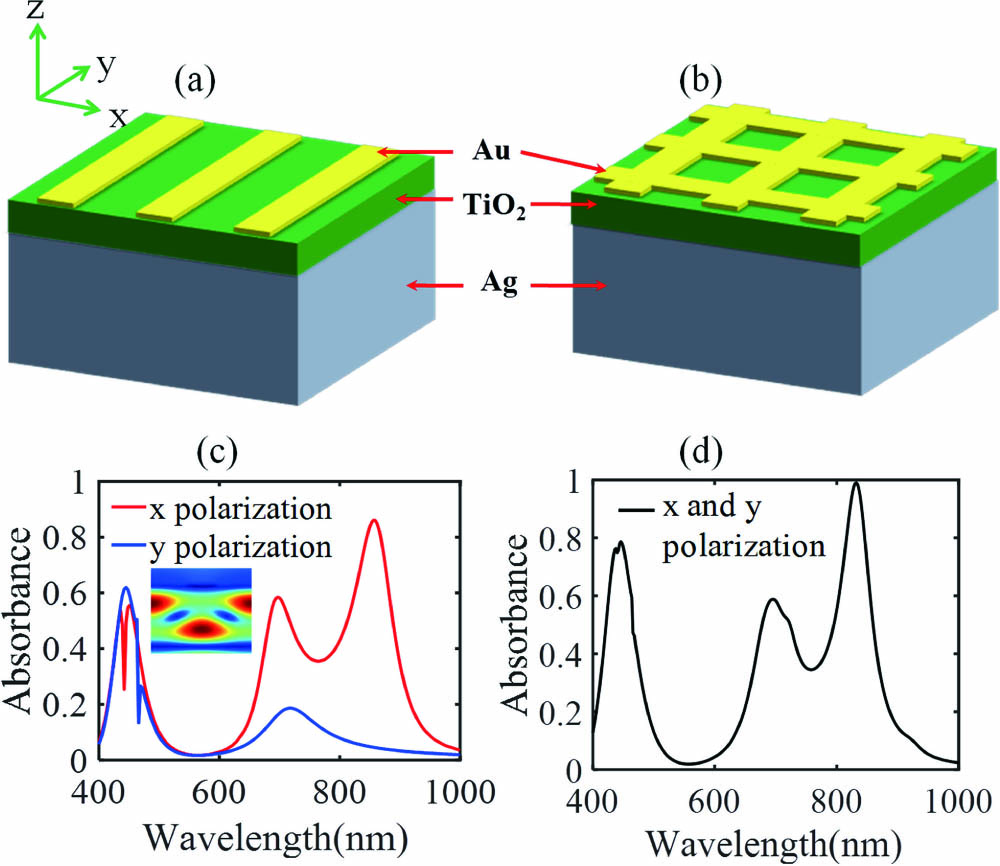
Fig. 8. Example showing reflectivity for the single-shock shot at 362 GPa (shot 302 in Table I in the Appendix ), as measured on each arm of the VISAR system, and assuming that the initial reflectivity is equal to the theoretical reflectivity of Al, equal to 88% (black dashed line). The reflectivity in the shocked region is equal to 53% ± 3%, with the uncertainty being due to the different values from each arm and to uncertainty in the fitting to the initial Al value. The gray dotted line indicates the maximum reflectivity expected from the QMD theoretical results.
The estimated temperatures are used for the plot in
4 IV. CONCLUSION
We have demonstrated that self-impedance mismatch has the capability to apply the results of Hugoniot measurements on plastics to directly determine conditions away from the principal Hugoniot. Such conditions are important for studying the behavior of materials under a much wider range of conditions, with particular relevance for planetary interiors, since single-shock compression to planetary-relevant pressures reaches far higher temperatures than are believed to exist within such bodies. At high shock pressures, our results seem to indicate a higher than expected reflectivity, and further work would be required to confirm this. At conditions away from the Hugoniot, the observed shock-front reflectivity is below that expected for Hugoniot points at the same pressure or density, but, using temperatures estimated from simulations, the reflectivities agree better. This may indicate that the metallization behavior is the same at both on- and off-Hugoniot states, with implications for planetary interiors, or may not hold true as the carbon demixes and forms diamond at later times. Future work with direct temperature measurement, such as by streaked optical pyrometry, would provide greater certainty about the EOS behavior under such double-shocked conditions and would serve as a benchmark for simulation codes.
Article Outline
N. J. Hartley, C. Zhang, X. Duan, L. G. Huang, S. Jiang, Y. Li, L. Yang, A. Pelka, Z. Wang, J. Yang, D. Kraus. Dynamically pre-compressed hydrocarbons studied by self-impedance mismatch[J]. Matter and Radiation at Extremes, 2020, 5(2): 028401.