Scenario of a magnetic dynamo and magnetic reconnection in a plasma focus discharge
1 I. INTRODUCTION
Fusion-interest magnetized plasmas, which are produced in Z-pinch and dense plasma-focus (PF) devices,
The existence of current flows and magnetic fields in the ordered plasma structures was explained based on the plasma density and plasma gradients inside the plasmoidal and toroidal structures that were calculated from the shifts of the recorded interferometric fringes using the Abel transformation.
In the present work, we discuss in more detail the possible evolution of the internal magnetic fields, based on the magnetic dynamo and magnetic reconnection effects, which have generally appeared in all the experiments conducted in the PF-1000 facility. This work may help to interpret the evolution of the ordered plasma structures recorded on the interferometric and extreme ultraviolet (XUV) frames and to understand the conditions that occur during the acceleration of fast electrons and deuterons. The phases in the formation of the plasmoid, in the stagnation of the pinch column, as well as in the evolution of instabilities and secondary plasmoids followed by a decay of the dense pinch column were observed on the frames recorded for all the discharges investigated. We have estimated the currents in the toroidal tube that surrounds the dense column during the stagnation phase. The device and diagnostics used in the experiments reported are described in Sec.
2 II. DESCRIPTION OF THE DEVICE AND DIAGNOSTICS
The experiments reported were performed with the PF-1000 plasma-focus facility, which was equipped with Mather-type coaxial electrodes of length 480 mm. The anode was a thick-wall copper tube of diameter 230 mm, and the cathode of diameter 400 mm consisted of 12 stainless-steel tubes (each of diameter 82 mm). The filling pressure of the pure deuterium was 170 Pa, and the main capacitor bank (charged up to 16 kV) provided the peak current of 1.2 MA–1.3 MA.
The voltage and current-derivative waveforms, as well as the total current intensity traces, were measured at the main collector plate. Time-resolved soft X-ray (SXR) signals were recorded with a silicon p-i-n diode shielded with a Be filter of thickness 10 µm. Three scintillation detectors were used to record signals at a distance of 7 m from the focus center in the downstream (at 0°), upstream (at 180°), and side-on (at 90°) directions. These measurements allowed us to determine the instants of the emission of hard X-rays (HXRs) and fusion-produced neutrons. The mean values of the energy of the fusion neutrons and primary deuterons were determined with the time-of-flight method from the temporal difference in the amplitudes of the neutron signals recorded downstream and upstream.
The total neutron yield, which was of the order of 1010–1011, was measured using two calibrated silver activation counters, which were placed outside the main experimental chamber. These counters were calibrated using an Am–Be source placed on the anode axis. In all cases, the maximum of the dip in the current derivative was assigned as the instant t = 0. This corresponded to the formation of the first plasmoid and a peak of the SXR signal. The time from the breakdown to the derivative dip was about 7 µs. The evolution of the constriction started at 50–100 ns. The formation of secondary plasmoids and the decay of the constriction was usually recorded 100 ns–200 ns after the dip. The uncertainty in the timing of different measuring channels during a single discharge was about 3 ns–5 ns.
3 III. EXPERIMENTAL RESULTS
3.1 A. Release of the discharge energy
The energy released in the plasma during the period Δt was calculated using the waveforms recorded for the voltage V and current I as the product UIΔt. For the average values of the voltage and current, which were 10 kV and 1 MA, respectively, it was estimated that the energy released was 100 J during each 10 ns evolution of the pinch. A higher proportion of the energy could be released during the voltage peak and the dip of the current derivative. This energy could be used to accelerate the plasma due to the Ampere force, generation of magnetic fields, and Joule heating. The Joule heating might increase the electron temperature and become lost through Bremsstrahlung radiation. The Ampere force accelerates the plasma-carrying current. The experiments described show that during the acceleration and implosion phase, only some of the discharge energy was transformed into the kinetic energy of the current sheath. Some of the energy was probably transformed into magnetic energy. The discharge current has a filamentary structure.
3.2 B. Filamentation of the current
The visible and XUV frames show that the current and the plasma sheath in many PF experiments had a filamentary character, during both the acceleration and implosion phases.
3.3 C. Dissipation of the magnetic field
The dissipation of the magnetic field in the plasma can be characterized by the magnetic Reynolds number Rm. Its value depends on the characteristic dimensions. Macroscopic plasma structures of 1 cm scale have a high Rm ≈ 103–104, which indicates that there is a strong frozen magnetic field with negligible dissipation for a longer time (at an electron temperature of about 50 eV above tens of microseconds). For filamentary plasma structures of size about several tens of micrometers, Rm is considerably smaller and indicates that the frozen and dissipated magnetic field components are in equilibrium. Consequently, in the filaments that exist during the acceleration and implosion of the current sheath, some of the kinetic energy (according to experimental estimations, about 50%) can be dissipated as magnetic energy. Then, the filamentary structures can explain the observed penetration of the magnetic field into the surrounding plasma in all phases of its evolution, i.e., during the motion of the current sheath, the pinch phase, the stagnation, the evolution of constrictions, and the decay of the dense pinch column.
3.4 D. Magnetic dynamo
A magnetic dynamo together with self-organization can explain the appearance of closed components of the magnetic field in PF-1000 discharges, which were analyzed using magnetic probe measurements.
The generation and transformation of the magnetic field, considered in this paper as being due to a magnetic dynamo,
The electron densities in the PF-1000 discharges were of the order of 1024 m−3–1025 m−3. The velocities of the plasma column transformations, as observed and described in Ref.
3.5 E. Self-generation of a magnetic field during the implosion of the current sheath
The appearance of self-generated closed azimuthal currents in PF-1000 plasmas was deduced from interferometric frames recorded during the radial implosion of the dense current sheath, and particularly from the closed dense interferometric fringes that form a toroidal structure. An example is shown in Fig.

Fig. 1. Interferometric frames from shot 12 586. (a) and (b) During the implosion. (c) and (d) During the formation of the plasmoid. (e) and (f) During the stagnation phase. In (a), the internal toroidal structures are within the solid white ellipses. In (b), the external toroidal structures are within the solid black ellipses. In (c), the maximal axial current flow in the column occurs within the dashed black ellipse. In (d), the internal plasmoidal structure is within the white dashed ellipse. In (e), the phase of the pinch column with rare fringes in its interior is within the black dashed ellipse.
The closed interferometric fringes show small toroidal and helical tubes formed by the dominant azimuthal current flow. This may be due to the dissipation of some current filaments, whose energy may be transformed into magnetic energy through the generation of magnetic turbulence. The turbulence develops into larger forms by spontaneous transformation accompanied by magnetic reconnections. Due to the α effect, the turbulence can induce an increase in the azimuthal current component and the corresponding poloidal magnetic field. During the acceleration and implosion of the plasma sheath, the azimuthal current can reach about 10% of the recorded axial current.
3.6 F. Transformation at the stopping of the imploded plasma sheath
At the current derivative dip, the implosion of the plasma sheath stops, but the current continues to penetrate into the dense plasma column
The initial phase of this toroidal-like tube formation is marked in
The axial plasma transport in the dense pinch column, which results in the stagnation and the plasmoid, may be caused by the higher magnetic pressure of the increasing azimuthal current in this part of the column boundary. Some estimates for the pressures and currents are presented in Sec.
3.7 G. Stagnation phase
During the stagnation phase [
![Interferometric frames from shot 12 605 recorded at different phases: [(a) and (b)] During the pinch stagnation. [(c), (d), and (e)] During the evolution of the constrictions. [(e) and (f)] During the decay of the plasma column structures. In (b), the black ellipse marks the profile of the toroidal tube and the dashed black line is the cross section that was used to calculate the closed currents.](/richHtml/mre/2020/5/4/046401/img_2.jpg)
Fig. 2. Interferometric frames from shot 12 605 recorded at different phases: [(a) and (b)] During the pinch stagnation. [(c), (d), and (e)] During the evolution of the constrictions. [(e) and (f)] During the decay of the plasma column structures. In (b), the black ellipse marks the profile of the toroidal tube and the dashed black line is the cross section that was used to calculate the closed currents.
![XUV frames from shot 12 605 for the same discharge shown in the interferometric images in Fig. 2. These were recorded at different phases: [(a) and (b)] During the pinch stagnation. [(c) and (d)] During the evolution of the pinch constrictions. These frames, unlike the interferometric images, show distinct helical structures at the surface of the dense plasma column and at the lobule top, which can be explained by an increase in the toroidal component after the pinch stagnation.](/richHtml/mre/2020/5/4/046401/img_3.jpg)
Fig. 3. XUV frames from shot 12 605 for the same discharge shown in the interferometric images in Fig. 2 . These were recorded at different phases: [(a) and (b)] During the pinch stagnation. [(c) and (d)] During the evolution of the pinch constrictions. These frames, unlike the interferometric images, show distinct helical structures at the surface of the dense plasma column and at the lobule top, which can be explained by an increase in the toroidal component after the pinch stagnation.
To estimate the currents and magnetic fields, we used the cross section [dashed line in
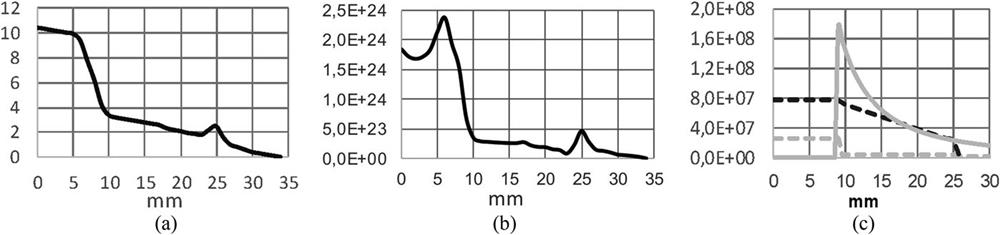
Fig. 4. Computation results: (a) Radial distribution of the interferometric fringes along the line of the cross section marked in Fig. 2(b) . (b) Radial distribution of the electron density calculated from the Abel transformation of the data shown in (a). (c) Distribution of the plasma pressure (dashed black line) and the magnetic pressure associated with the pinching poloidal current I p (full gray line) and the repulsive toroidal current I t (dashed gray line).
Considering both magnetic field components, the ratio of the plasma and magnetic pressures β in that case was about 1. The final current It ≈ 180 kA was larger than the initial current of about 70 kA ≈ 10% of Iz, evidently due to the self-generation effect. The total energy, which was contained in a volume of diameter 2 cm, could reach kilojoules.
3.8 H. Evolution of constriction and secondary plasmoids
In the final phases of the pinch stagnation in PF-1000 discharges, one can usually observe some stratification on the surface of the plasma column, as shown in
3.9 I. Release of the magnetic energy and acceleration of fast electrons and ions
The dominant HXR and neutron production usually starts during the decay of the plasmoids and the disruption of the constriction. The narrow constriction often decays axially when it is absorbed into the secondary plasmoids or by the formation of new third-generation plasmoids, as one can see in
The tracks of fast deuterons recorded end on
The length of the plasma column usually increases linearly with the volume of the closed currents. The magnetic field B decreases as 1/r (at the radial distribution of that), and when the current is 1 MA, it reached B ≈ 0.2/r, where r is the radius expressed in meters. Then, the magnetic energy also increases linearly with r, and its total value in the volume above the anode front can reach several kilojoules (for several hundreds of nanoseconds). This energy can be contained in the closed local magnetic fields and it can be released as energetic particle beams, which are emitted from the plasma column (mainly during its decay), as well as from its surroundings.
This energy of about several kilojoules was estimated from the measured neutron yield, the mean energy of the accelerated fast deuterons, the cross section of D–D fusion reactions for this deuteron energy, and the known density of the dense plasma at the top of the dense pinch column. The mean energy of fast deuterons was estimated from the temporal differences in the neutron signals recorded in the downstream and upstream directions. The mean energy can reach 5 kJ. The detailed calculations were presented in an earlier paper.
Characteristic waveforms and fast neutron signals were recorded for almost all investigated PF-1000 discharges. Some examples are presented in
![Waveforms from shot 12 605. Gray: The current derivative with a dip. Green: Voltage with a peak at the smallest pinch diameter. Yellow: SXR pulses emitted at the current derivative dip, at the voltage peaks, and at the formation of the first plasmoid. Red: HXR signal. Blue: Neutrons at 2.45 MeV shifted in time. The neutrons begin to be emitted during the disruption of the constriction [Fig. 2(e)]. The SXRs increase during the transformation of the kinetic energy into heat.](/richHtml/mre/2020/5/4/046401/img_5.jpg)
Fig. 5. Waveforms from shot 12 605. Gray: The current derivative with a dip. Green: Voltage with a peak at the smallest pinch diameter. Yellow: SXR pulses emitted at the current derivative dip, at the voltage peaks, and at the formation of the first plasmoid. Red: HXR signal. Blue: Neutrons at 2.45 MeV shifted in time. The neutrons begin to be emitted during the disruption of the constriction [Fig. 2(e) ]. The SXRs increase during the transformation of the kinetic energy into heat.
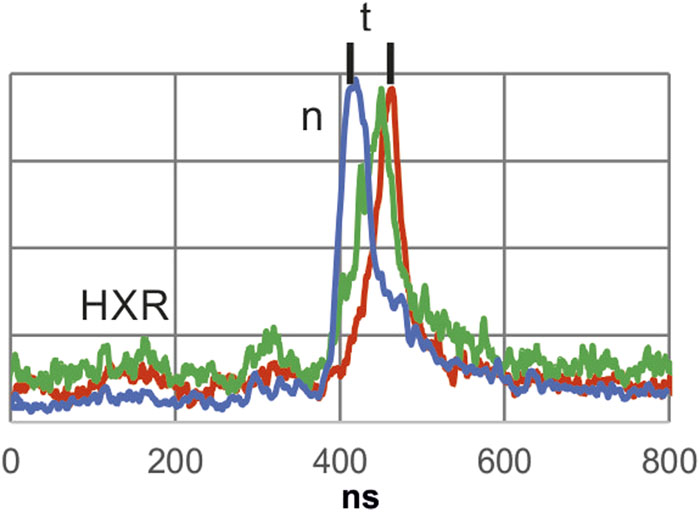
Fig. 6. Neutron signals recorded at a distance of 7 m in the downstream (blue), side-on (green), and upstream (red) directions. The temporal shift of the peaks (t ) made it possible to calculate the dominant neutron velocity (downstream and upstream) and the mean energy E d of the deuterons producing the fusion neutrons. In this shot, it was estimated that E d ≈ 100 keV at t ≈ 50 ns. In all these PF-1000 shots, E d reached values of 70 keV–500 keV. At the mean deuteron energy of 200 keV, the increase in the FWHM of the neutron pulse (measured at 7 m) was about 20 ns.
3.10 J. Discussion on the acceleration of fast deuterons
Schmidt et al.
A possible explanation for the formation of the organized plasma structures is described in a new paper.
Recently, the ion acceleration mechanism has been studied in detail during experiments performed with the GIT-12 generator. Deuterium gas-puffed Z-pinch discharges produced ions with energies of up to 30 MeV.
4 IV. SUMMARY
In this paper, we presented the transformations of plasma energy during the evolution of the ordered plasma structures that are visible in interferometric frames. The magnetic dynamo effect is a possible mechanism. The α effect may occur during fluctuations of plasma densities and velocities, as well as changes in the local magnetic fields of the current filaments, which have diameters of several tens of micrometers. At this scale, the plasma is collision-less and the magnetic Reynolds number is close to 1, which allows the magnetic energy to dissipate quickly. During this transformation, plasma streams can interact with the magnetic field to form magnetic turbulence, which can spontaneously generate, through magnetic reconnections, ordered plasma structures with closed internal currents and corresponding magnetic fields. An example was calculated from the distribution of the electron densities during the stagnation phase. This process can accumulate an energy of several kilojoules in the plasma volume in front of the anode. Most of this energy can subsequently be released from this volume in a relatively short time of several tens of nanoseconds in the form of the observed HXR and fusion neutrons.
The results presented are evidently qualitative. Their verification and a more exact quantitative description of the energy transfer needs further experimental studies supported by appropriate numerical simulations.
[1] A. Bernard, H. Bruzzone, P. Choi, et al.. Scientific status of plasma focus research. ., 1998, 8: 93.
[4] M. G. Haines. A review of the dense Z-pinch. Plasma Phys. Controlled Fusion, 2011, 53: 093001.
[11] L. Karpinski, M. Paduch, M. Scholz, et al.. Recent progress in 1 MJ Plasma-Focus research. Nukleonika, 2001, 46: 35.
[12] M. J. Sadowski, M. Scholz. Results of large-scale Plasma-focus experiments and prospects for neutron yield optimization. Nukleonika, 2002, 47: 31.
[16]
[19] H. Ji, R. Kulsrud, M. Yamada. Magnetic reconnection. Rev. Mod. Phys., 2010, 82: 603.
[24] W. H. Bostic. The pinch effect revisited. Int. J. Fusion Energy, 1977, 1: 1.
[26] B. A. Trubnikov. Current filaments in plasmas. Plasma Phys. Rep., 2002, 28: 312.
[27]
[31] P. Kubes, M. Paduch, M. J. Sadowski, et al.. External current layer of a pinch column in a dense plasma focus. Phys. Plasmas.
Article Outline
P. Kubes, M. Paduch, M. J. Sadowski, J. Cikhardt, D. Klir, J. Kravarik, R. Kwiatkowski, V. Munzar, K. Rezac, A. Szymaszek, K. Tomaszewski, E. Zielinska, M. Akel, B. Cikhardtova. Scenario of a magnetic dynamo and magnetic reconnection in a plasma focus discharge[J]. Matter and Radiation at Extremes, 2020, 5(4): 046401.