Pressure responses of halide perovskites with various compositions, dimensionalities, and morphologies
Download: 501次
1 I. INTRODUCTION
In the past several years, photovoltaic devices based on halide perovskites (HPVs) have made impressive progress in their development, attaining energy conversion efficiencies of over 25%, surpassing the already commercialized polysilicon, cadmium telluride, and copper indium gallium selenide photovoltaic devices.
Despite their unique properties and impressive achievements, inherent challenges remain in HPVs that need to be addressed, calling for a better understanding of the mechanisms that dominate their outstanding functionalities. The soft lattices of HPVs render them sensitive to mechanical compression, which can effectively adjust their atomic and electronic structures, as well as their physical properties, without changing their chemical composition. In combination with various in situ characterization probes, high-pressure research could further our fundamental understanding of the structure-property relationship. As a fundamental parameter, pressure can efficiently adjust the structures of materials, leading to exceptional enhancements from external dimensional tuning. In this review, we summarize the recent progress made in the research of pressure-induced changes in the structures and properties of HPVs. We begin by introducing the characteristics of HPVs and the fundamental knowledge of high-pressure techniques together with in situ characterizations. We then discuss the effects of pressure on HPVs with different compositions, dimensionalities, and morphologies, focusing on the enhanced and emergent properties induced by high-pressure treatment, as well as the structure-property relationship. The final section highlights the main challenges and outlook of high-pressure research on HPVs.
1.3 A. Crystal structures and physical properties of HPVs
Perovskite is a class of materials that has the general formula ABX3.
![(a) Schematic diagram of perovskite structure. This figure is reproduced with permission from Yin et al., J. Mater. Chem. A 3, 8926–8942 (2015). Copyright 2015 The Royal Society of Chemistry.28 (b) Tolerance factors (t) for different organic–inorganic HPVs. This figure is reproduced with permission from Fan et al., J. Mater. Chem. A 3, 18809–18828 (2015). Copyright 2015 The Royal Society of Chemistry.21 (c) Crystal structures of 3D and 2D HPVs. This figure is taken from Ref. 27. (d) Crystal structures of the 2D HPV of L2An-1BnX3n+1 (from n = 1 to n = ∞) with different [BX6]4− octahedral layers. The number of inorganic layers n = ∞ corresponds to 3D HPV structure. (c) and (d) Reproduced with permission from Jaffe et al., ACS Energy Lett. 2, 1549–1555 (2017). Copyright 2017 American Chemical Society.27](/richHtml/mre/2020/5/1/018201/img_1.jpg)
Fig. 1. (a) Schematic diagram of perovskite structure. This figure is reproduced with permission from Yin et al. , J. Mater. Chem. A 3 , 8926–8942 (2015). Copyright 2015 The Royal Society of Chemistry.28 (b) Tolerance factors (t ) for different organic–inorganic HPVs. This figure is reproduced with permission from Fan et al. , J. Mater. Chem. A 3 , 18809–18828 (2015). Copyright 2015 The Royal Society of Chemistry.21 (c) Crystal structures of 3D and 2D HPVs. This figure is taken from Ref. 27 . (d) Crystal structures of the 2D HPV of L2An -1Bn X3n +1 (from n = 1 to n = ∞) with different [BX6]4− octahedral layers. The number of inorganic layers n = ∞ corresponds to 3D HPV structure. (c) and (d) Reproduced with permission from Jaffe et al. , ACS Energy Lett. 2 , 1549–1555 (2017). Copyright 2017 American Chemical Society.27
The earliest research conducted on organic–inorganic lead HPVs (e.g., MAPbX3, X = Cl, Br, and I) mainly focused on their outstanding photovoltaic performance. All inorganic compounds (e.g., CsPbX3) then became attractive because of their high stability and tunable functionalities. Both theoretical and experimental evidence has shown that the three elements of A, B, and X in the ABX3 crystalline structure can be chemically substituted, resulting in a series of mixed compounds.
More recently, the reduced-dimensional HPVs, especially two-dimensional (2D) layered perovskite materials, have given rise to a very diverse semiconductor subgroup with high tunability and eminently adjustable photophysical properties.
HPVs are typically direct bandgap materials with high optical absorption coefficients. The bandgap of a semiconductor determines the absorption width of the solar spectrum, and HPVs exhibit a bandgap ranging from about 1 to 3 eV. For 3D HPVs, most of their bandgap edges consist of orbitals from metal B and halogen X.
The interaction among metal-halide units in 3D HPVs results in the formation of electron bands that have large bandwidths. In addition to possessing a long distance for carrier transport, HPVs absorb a wide range of wavelengths, possessing a high absorption coefficient, producing an outstanding photovoltaic performance.
The bandgaps of the materials can be optimized by different methods, influencing optoelectronic performance. One possible way to adjust the bandgap of the material is to change the chemical composition or doping of the material, such as by changing the proportion of constituent halides. The mixed-cation HPVs with lead and tin at the B sites can also reduce the bandgap, but this shortens their carrier lifetime and compromises their stability.
1.4 B. Basic knowledge of high-pressure science and technology
As a thermodynamic parameter, pressure can be employed to tune the properties of materials by adjusting their interatomic distances, electronic orbitals, and bonding patterns.
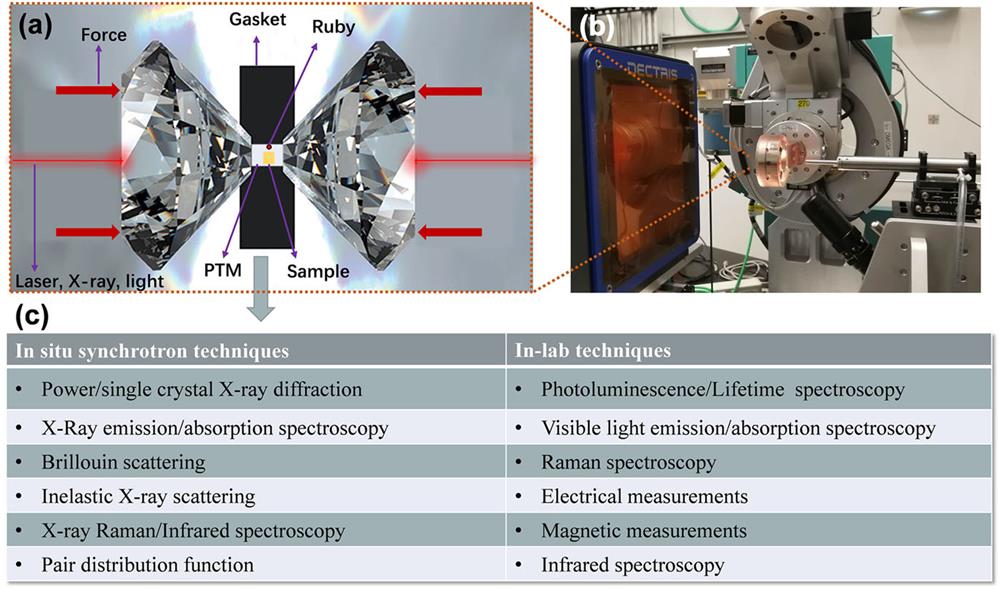
Fig. 2. (a) Schematic diagram of the sample in a DAC. (b) High-pressure synchrotron-based setup for diffraction. (c) In situ in-laboratory and synchrotron-based characterization tools under high pressures.
In the last several years, the previously small field of high-pressure science and technology has grown significantly, becoming increasingly attractive to the physics, chemistry, and materials sciences communities.
The study of HPVs under high pressure can be traced back to the 1990s and focused on their P-T phase diagrams of MAPbX3.
2 II. PRESSURE-TUNED AND PRESSURE-ENHANCED PROPERTIES
In general, pressure-induced shortening of the bond length narrows the bandgap while bending of the B–X–B angle widens it.
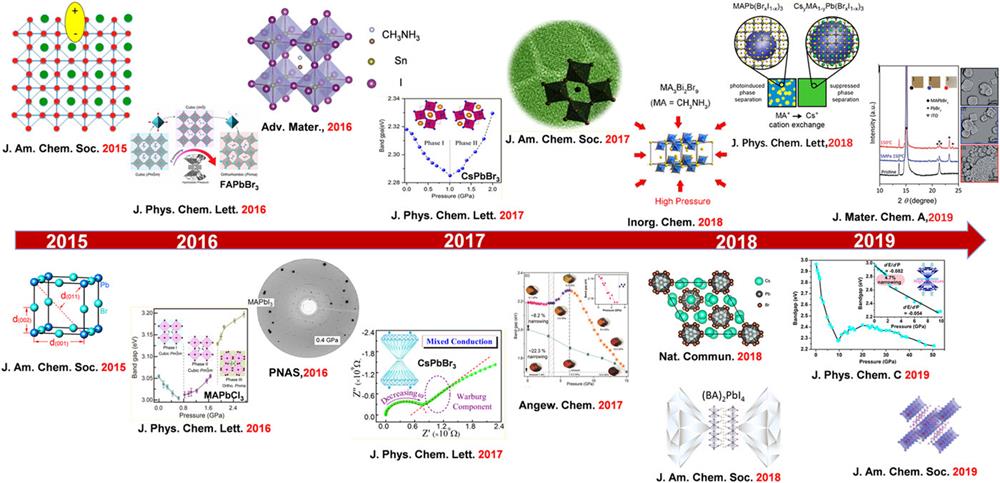
Fig. 3. The research progress of HPVs under high pressure. Reproduced with permission from Wu et al., J. Am. Chem. Soc. 137 , 2089–2096 (2015). Copyright 2015 The Royal Society of Chemistry;33 Wang et al., J. Am. Chem. Soc. 137 , 11144–11149 (2015). Copyright 2015 The Royal Society of Chemistry;54 Wang et al., J. Phys. Chem. Lett. 7 , 2556–2562 (2016). Copyright 2016 The Royal Society of Chemistry;86 Wang et al., J. Phys. Chem. Lett. 7 , 5273–5279 (2016). Copyright 2016 The Royal Society of Chemistry;87 Lu et al., Adv. Mater. 28 , 8663–8668 (2016). Copyright 2016 Wiley-VCH Verlag GmbH & Co. KGaA;37 Kong et al., Proc. Natl. Acad. Sci. 113 , 8910–8915 (2016);82 Zhang et al., J. Phys. Chem. Lett. 8 , 3752–3758 (2017). Copyright 2017 The Royal Society of Chemistry;84 Yan et al., J. Phys. Chem. Lett. 8 , 2944–2950 (2017). Copyright 2017 The Royal Society of Chemistry;70 Xiao et al., J. Am. Chem. Soc. 139 , 10087–10094 (2017). Copyright 2017 The Royal Society of Chemistry;43 Wang et al., Angew. Chem., Int. Ed. 56 , 15969–15973 (2017). Copyright 2017 Wiley-VCH Verlag GmbH & Co. KGaA;83 Zhu et al., Inorg. Chem. 57 , 6206–6209 (2018). Copyright 2018 The Royal Society of Chemistry;6 Ma et al., Nat. Commun. 9 , 4506 (2018). Copyright 2018 Springer Nature;11 Bischak et al., J. Phys. Chem. Lett. 9 , 3998–4005 (2018). Copyright 2018 The Royal Society of Chemistry;5 Ding et al., J. Mater. Chem. A 7 , 540–548 (2019). Copyright 2019 The Royal Society of Chemistry;20 Ren et al., J. Phys. Chem. C 123 , 15204–15208 (2019). Copyright 2019 The Royal Society of Chemistry;88 and Shi et al., J. Am. Chem. Soc. 141 , 6504–6508 (2019). Copyright 2019 The Royal Society of Chemistry.85
2.3 A. 3D organic–inorganic HPVs
3D organic–inorganic HPVs are the type studied most and they exhibit the best photovoltaic performance. In particular, the electronic structures and optical properties of MAPbX3 under pressure have been experimentally and theoretically investigated.
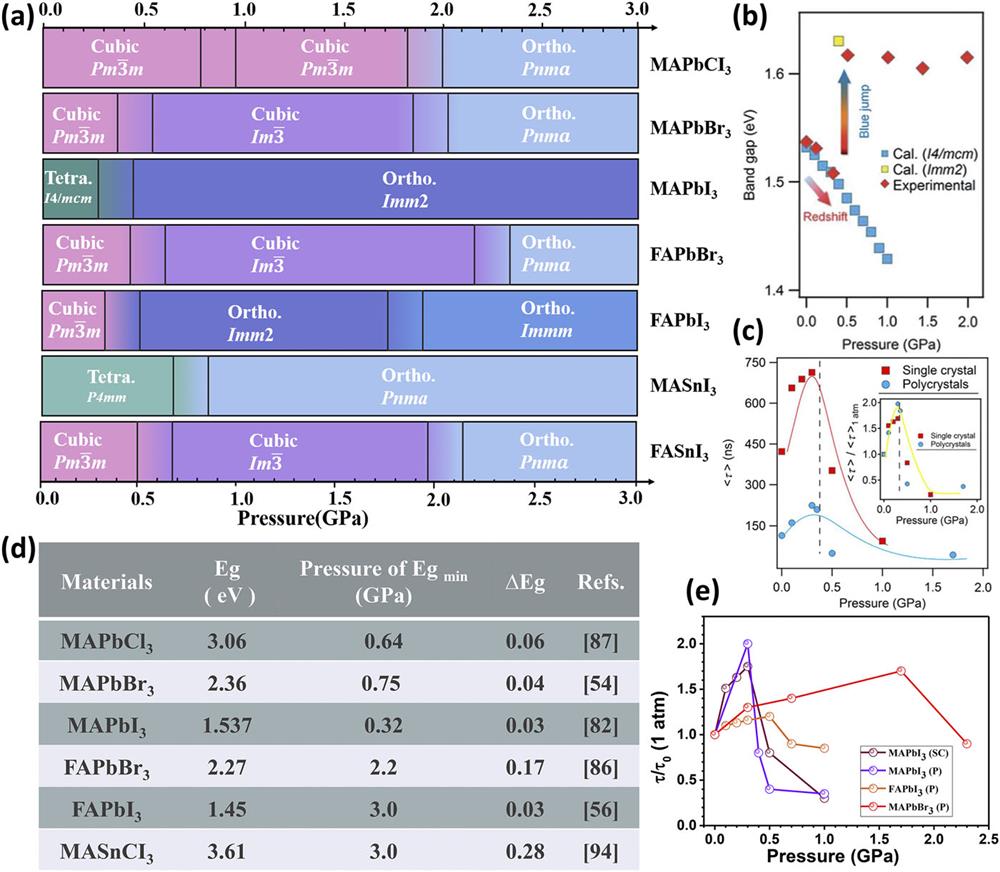
Fig. 4. (a) Summary of pressure-induced structural evolution in MAPb/SnX3. (b) Pressure-induced bandgap evolution of MAPbI3. (c) Pressure dependence of average carrier lifetimes of single crystal and polycrystalline MAPbI3; inset shows normalized results. (b) and (c) Reproduced with permission from Kong et al., Proc. Natl. Acad. Sci. 113 , 8910–8915 (2016).82 (d) Comparison of pressure-induced variations of the electronic structure of MA/FAPbX3. These include the bandgap (Eg), the pressure at the minimum bandgap (Egmin), and the bandgap decreased values (∆Eg). These figures are taken from Refs. 54 , 56 , 82 , 86 , 87 , and 94 . (e) Summary of several organic–inorganic HPV carrier lifetimes under high pressure.
It is inevitable that pressure-induced structural transitions lead to variations in bandgaps. According to the so-called Shockley–Queisser theory, the optimum bandgap for solar cells is 1.34 eV, which offers a theoretical power conversion efficiency of 33%. However, the bandgaps of HPVs are normally greater than this ideal value. In situ high-pressure optical measurements, such as absorption spectroscopy and PL spectroscopy, could be used to monitor and understand the bandgap evolution of HPVs. During compression, the electron distribution and orbital interactions within the system can be changed by decreasing interatomic distances, thus adjusting the band structures. For the organic–inorganic HPVs with [BX6]4− octahedra, the pressure response of the bandgaps is between 17 and 100 meV/GPa. Kong et al. achieved a simultaneous evolution of bandgap narrowing (0.03 eV) and PL lifetime prolongation of single MAPbI3 crystals under a pressure of 0.3 GPa.
Electrical conductivity and photocurrent are important characteristics for applications in optoelectronic devices. High-pressure studies on the electrical properties of HPVs are critical to revealing structure-property relationships. Wang et al. conducted electrical resistance measurements on MAPbBr3 using a four-probe method within a DAC [
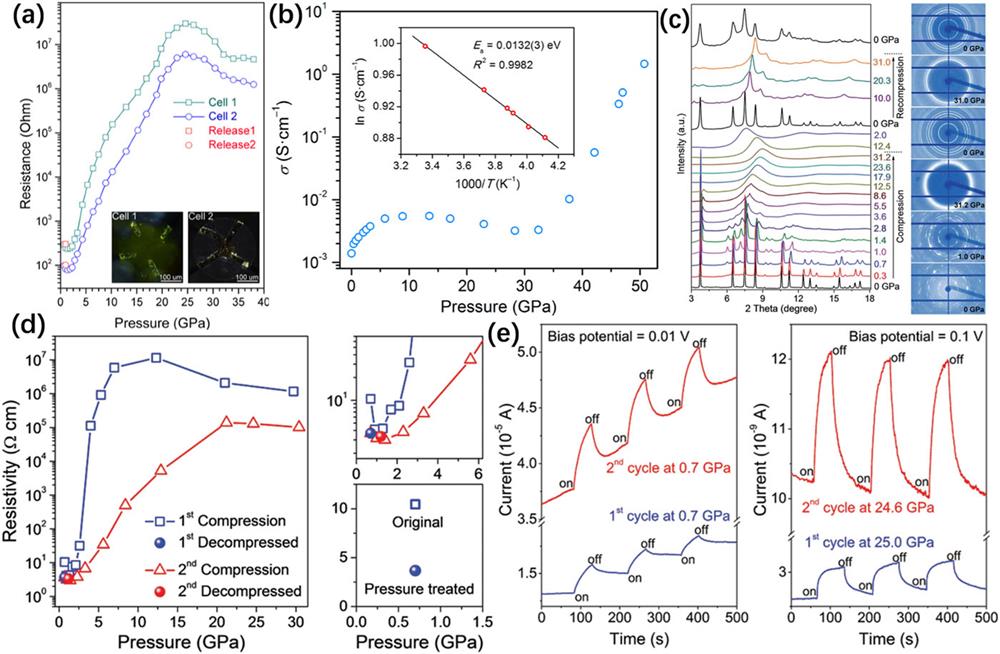
Fig. 5. (a) Electrical resistance as a function of pressure for MAPbBr3. The inset in panel (a) shows microphotographs of the samples with four Au probes in two DACs. This figure is reproduced with permission from Wang et al. , J. Am. Chem. Soc. 137 , 11144–11149 (2015). Copyright 2015 American Chemical Society.54 (b) Electrical conductivity of MAPbI3 as a function of pressure. Inset shows the Arrhenius fit of the temperature-dependent conductivity at 51 GPa, which gives an activation energy Ea of 13.2(3) meV. This figure is taken from Ref. 91 . (c) XRD patterns of MASnI3 under cyclic pressurization and original XRD images at six selected pressures. (d) Pressure-induced evolution of electrical resistivity and comparison of resistivity before and after high-pressure treatment. (e) Photocurrent changes under pressure. The blue line indicates the first loop and the red line indicates the second loop. These figures are reproduced with permission from Lu et al. , Adv. Mater. 28 , 8663–8668 (2016). Copyright 2016 Wiley-VCH Verlag GmbH & Co. KGaA.37
Lu et al. systematically investigated the differences in the structures and properties of lead-free HPV before and after high-pressure treatments.
From these high-pressure investigations, one can optimize the performance of the 3D HPVs via introducing artificial stress and strain. Here, based on the pressure-induced changes in the crystal structure, lifetime, electrical resistance, and photocurrent of organic–inorganic HPVs, we underline some general conclusions and common features: Pressure-induced structural transitions and further amorphization occur in 3D organic–inorganic HPVs, usually in the order of a few gigapascals, owing to their soft lattices and low bulk modulus; The electronic bandgap usually narrows by pressure-induced lattice contraction and widens by increasing octahedral tilting angles or amorphization; Electrical resistance can increase by two to five orders of magnitude during compression.
2.4 B. 3D inorganic cesium lead HPVs
The substitution of organic cations (MA+ and FA+) by inorganic Cs+ is a direct and effective way to enhance the stability of HPVs.
Table 1. Pressure-driven evolution of structure and bandgap in bulk and nanostructured inorganic HPVs, including the pressure values of crystal structural transition Eg, the pressure values of phase transition ∆Eg, the pressure at the minimum bandgap Egmin, and the pressure at initial amorphization and the bulk modulus (K0/B0).
|
Nagaoka et al. investigated the pressure-induced behavior of CsPbBr3 nanocube superlattices (NC-SLs).

Fig. 6. (a) WAXS patterns of CsPbBr3 nanocubes during compression and decompression. (b)–(d) Integrated WAXS spectra with calculated Bragg reflection positions at 1.4 GPa, 14.5 GPa, and total release of pressure, respectively. The bars represent the calculated Bragg reflection positions. (e) TEM image of the pressure-sintered NPLs. Inset shows the high-resolution (HR) TEM image of the pressurized sample with a lamellar structure before disassembly. (f) An HRTEM image and the corresponding FFT pattern (inset) of the pressure-synthesized CsPbBr3 NPLs. (g) Schematic demonstration of the pressure-sintering process: NC-SL evolution (top) and interparticle fusion (bottom). (h) Plots of the PL peak position of the NC-SL PLs (black) and the relative PL intensity (gray) as a function of pressure. The open square shows the PL intensity after decompression. These figures are reproduced with permission from Nagaoka et al. , Adv. Mater. 29 , 1606666 (2017). Copyright 2017 Wiley-VCH Verlag GmbH & Co. KGaA.98
Xiao et al. studied the pressure response of CsPbBr3 with various morphologies (nanocrystals, nanowires, and bulk materials), where simultaneous carrier lifetime prolongation and bandgap narrowing were observed.
2.5 C. Low-dimensional HPVs
Low-dimensional HPVs, possessing intrinsic quantum confinement effects, are promising for advanced photovoltaic and optoelectronic applications, especially for 2D layered compounds.
Liu et al. reported bandgap narrowing (633 meV) in an RP-type perovskite (BA)2(MA)Pb2I7 under pressure.
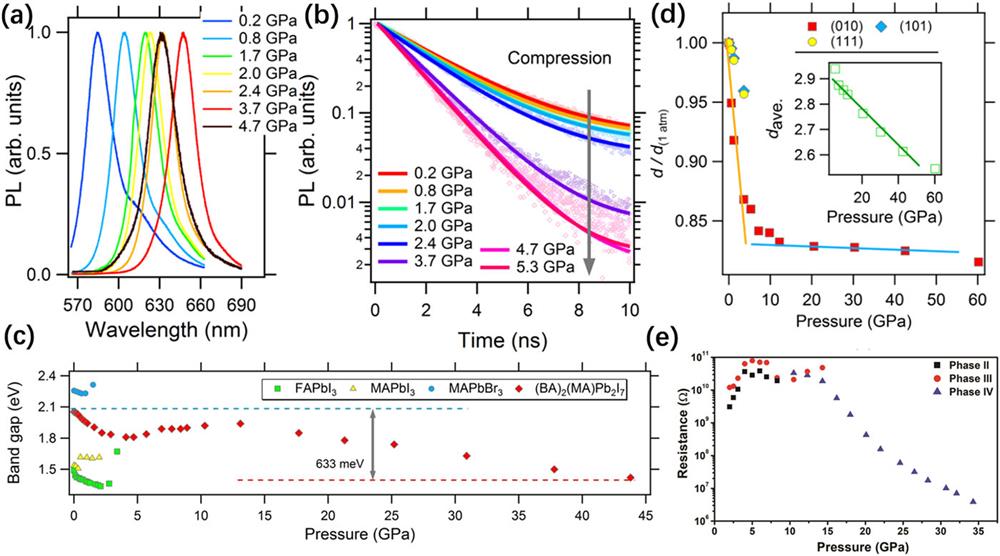
Fig. 7. (a) and (b) In situ static PL and time-resolved PL spectra of (BA)2(MA)Pb2I7 under high pressure. (c) Pressure-induced evolution of bandgap of (BA)2(MA)Pb2I7 and three 3D HPVs. (d) Pressure dependence of the relative changes in the d -spacing. These figures are reproduced with permission from Liu et al. , ACS Energy Lett. 2 , 2518–2524 (2017). Copyright 2017 American Chemical Society.112 (e) Pressure-induced evolution of the electrical resistance of (C4H9NH3)2PbI4. This figure is taken from Ref. 113 .
Yin et al. focused on the optical properties of mechanically exfoliated nm-thin flakes of 2D perovskite (C4H9NH3)2PbI4.
C4N2H14SnBr4 is a typical 1D HPV, in which the edge-sharing octahedral [SnBr4]2− anions are embraced by the organic [C4N2H14]2+ cations, serving as an assembly of core-shell quantum wires. Shi et al. reported an emergent emission of C4N2H14SnBr4 at pressures over ∼2 GPa.
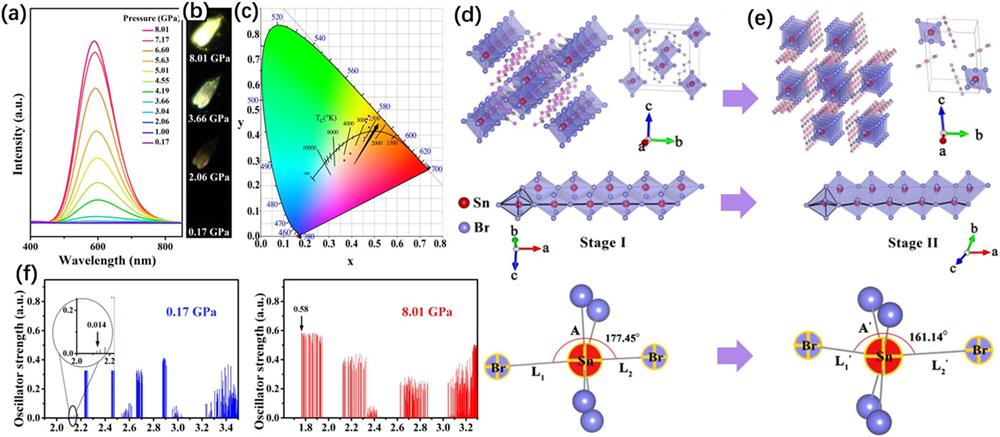
Fig. 8. (a) Pressure-dependent PL spectra of 1D C4N2H14SnBr4. (b) PL images of C4N2H14SnBr4 under different pressures. (c) Pressure-dependent chromaticity coordinates. (d) and (e) Crystal structures and Br–Sn–Br bond length and angle of C4N2H14SnBr4 before and after the structural transition. (f) Calculation of the absorption oscillator strengths using the excited-state structure associated with STEs at 0.17 and 8.01 GPa. These figures are reproduced with permission from Shi et al. , J. Am. Chem. Soc. 141 , 6504–6508 (2019). Copyright 2019 American Chemical Society.85
In addition, Ma et al. reported that nonfluorescent 0D inorganic HPV Cs4PbBr6 nanocrystals exhibit strong emission under high pressure.
2.6 D. Other novel HPVs
The halide-perovskite community continues to search for nontoxic, stable, and high-performance perovskite-like derivatives for photovoltaic and optoelectronic applications. Volonakis et al. reported the computational design and experimental synthesis of a new family of Pb-free inorganic halide double perovskites, such as Cs2BiAgCl6, materials that exhibit very promising optoelectronic properties, including tunable bandgaps in the visible range and low carrier effective masses.
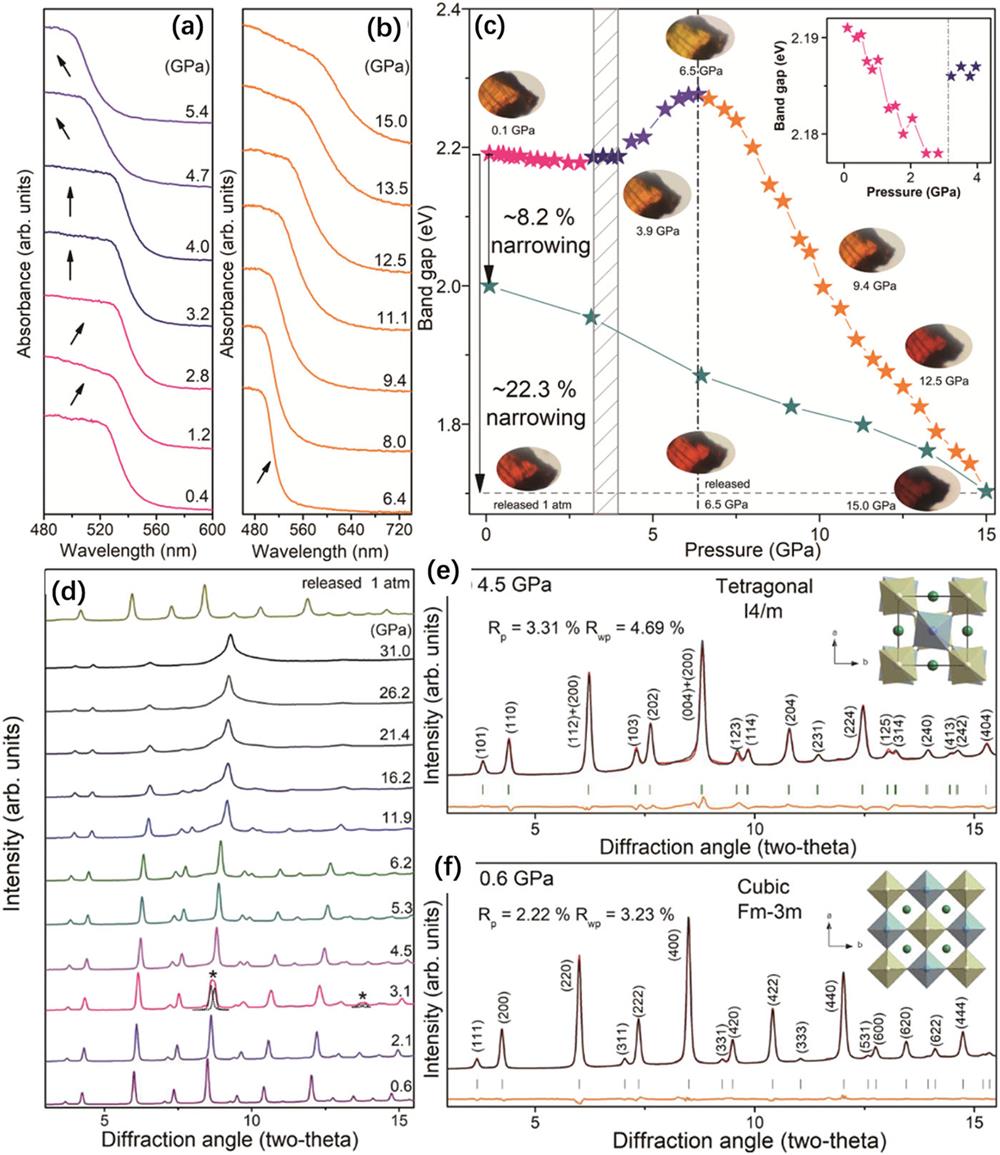
Fig. 9. (a) and (b) UV-Vis absorption spectra of Cs2AgBiBr6 under high pressure. (c) Pressure-induced bandgap evolution of Cs2AgBiBr6, and representative optical micrographs. (d) Angle-dispersive synchrotron XRD patterns of Cs2AgBiBr6 at selected pressures. (e) and (f) Rietveld refinements of angle-dispersive synchrotron XRD patterns recorded at 0.6 and 4.5 GPa, respectively. These figures are reproduced with permission from Li et al. , Angew. Chem., Int. Ed. 56 , 15969–15973 (2017). Copyright 2017 Wiley-VCH Verlag GmbH & Co. KGaA.83
Bounos et al. investigated the pressure-induced evolution of the structures and properties of defective perovskite Cs2SnX6 (X = Cl, Br, and I).
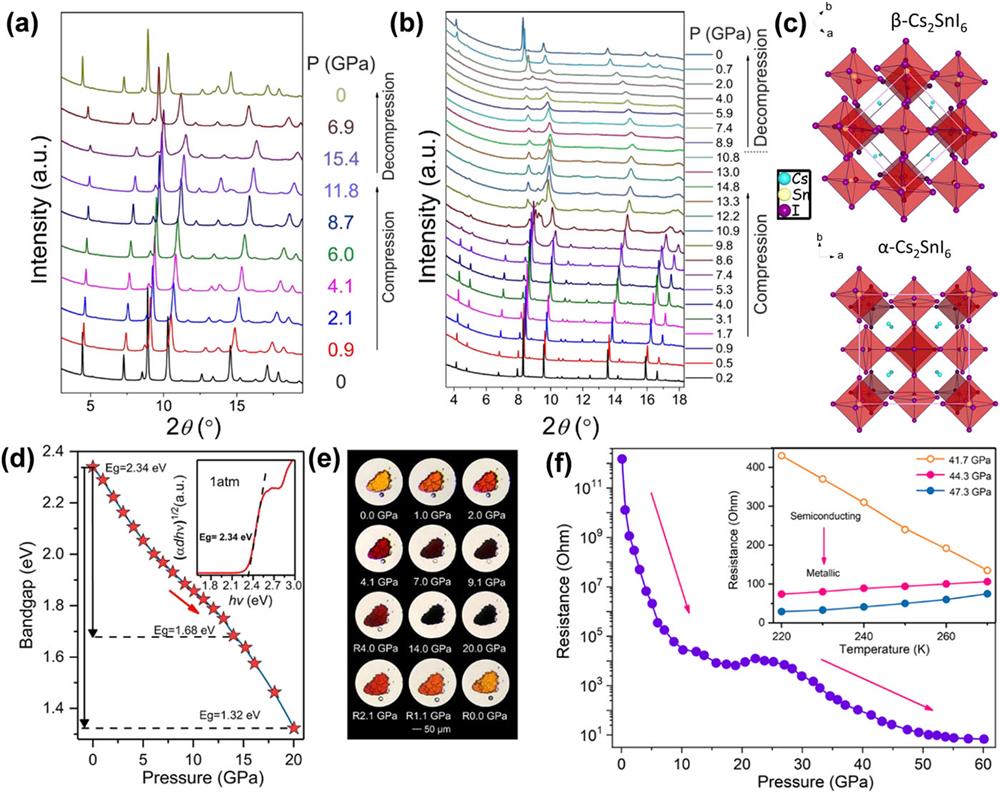
Fig. 10. High-pressure XRD patterns of (a) Cs2SnBr6 and (b) Cs2SnI6. (c) Two different crystal structures of Cs2SnI6, where the ambient pressure α -phase can be described as a body-centered tetragonal (BCT) unit cell with a lattice constant ratio , while the high-pressure β -phase (I 2/m ) can be derived from the ambient BCT structure through the tilting of the SnI6 octahedra along the b axis. These figures are reproduced with permission from Bounos et al. , J. Phys. Chem. C 122 , 24004–24013 (2018). Copyright 2018 American Chemical Society.117 (d) Bandgap evolution of Cs3Sb2I9 at various pressures. The inset shows the bandgap Tauc plot of Cs3Sb2I9 at 1 atm. (e) Optical micrographs of Cs3Sb2I9 during compression. (f) The electrical resistance of Cs3Sb2I9 under high pressure. The inset shows the resistance-temperature diagram at different pressures. At 44.3 GPa, the electrical resistance of Cs3Sb2I9 increases with temperature ramping, suggesting the metallic behavior. These figures are reproduced with permission from Wu et al. , ChemSusChem 12 , 3971–3976 (2019). Copyright 2019 Wiley-VCH Verlag GmbH & Co. KGaA.118
The structural manipulation and property enhancements achieved by high-pressure treatment provide motivation for the further exploration and modification of the optoelectronic properties of HPVs, with various compositions, dimensionalities, and morphologies. By integrating high-pressure techniques and in situ characterization methods, one can systematically investigate the pressure-induced variation of structures and properties. Furthermore, the conclusions obtained from high-pressure research offer a new perspective for a fundamental understanding of the relationships among the different compositions, structures, dimensionalities, morphologies, and optoelectronic properties of HPVs, providing the potential for optimization of these materials for future photovoltaics and optoelectronics.
3 III. SUMMARY AND OUTLOOK
HPVs have been extensively investigated for their unique properties and potential applications in photovoltaic and optoelectronic devices. Perovskite derivatives, with various compositions, dimensionalities, and morphologies, have been developed, which show superior properties, such as better stability and wider tunability. Impressive progress has recently been made in high-pressure research concerning this family of materials. In this paper, we have summarized this progress, where the pressure-induced variations of structural, optical, electrical, and optoelectronic properties are discussed. We pay particular attention to the enhanced and emergent properties induced by pressure and the structure-property relationship. Despite these achievements, high-pressure research on HPVs still faces a number of challenges: (1) A solid understanding of HPVs is still far from satisfactory, which is partly the result of limited in situ characterization methods, especially for real devices; (2) The small samples used (usually at the microscale) can introduce issues of nonuniformity and increased complexity, increasing uncertainty; (3) The intrinsic sensitivity of HPVs to light and moist air cause irreversible degradation and introduce difficulties in obtaining accurate data.
Future high-pressure research on HPVs should include: (1) Achieving a deeper understanding of the structure-property relationship in a more comprehensive manner with the help of more systematic characterizations. For example, revealing the nature of pressure-induced amorphous or disordered structures is particularly interesting for understanding emergent properties at high pressure. To this end, pair distribution function analyses of synchrotron x-ray and neutron total scattering data can be used to explore the characteristics of local structures. (2) Alternative routes can be explored to simulate the high-pressure effects of DACs to realize unique structures and properties, e.g., by using a large-volume press, chemical tailoring, and interfacial engineering. (3) Developing theoretical simulations based on experimental results will provide mechanistic explanations and thus further our fundamental understanding.
[7] , et al.. Perovskite LEDs. Sci. Video Protocols, 2019, 1: 1-5.
[9] , et al.. Advances in perovskite solar cells. Adv. Sci., 2016, 3: 1500324.
[10] . Toward lead-free perovskite solar cells. ACS Energy Lett., 2016, 1: 1233-1240.
[17] . What defines a perovskite?. Adv. Energy Mater., 2018, 8: 1802366.
[26] , et al.. Low-dimensional organometal halide perovskites. ACS Energy Lett., 2017, 3: 54-62.
[33] , et al.. Trap states in lead iodide perovskites. J. Am. Chem. Soc., 2015, 137: 2089-2096.
[40] , et al.. Materials discovery at high pressures. Nat. Rev. Mater., 2017, 2: 17005.
[46] . Ultrahigh-pressure transitions in solid hydrogen. Rev. Mod. Phys., 1994, 66: 671-692.
[47] . Photovoltaic hybrid perovskites under pressure. J. Phys. Chem. Lett., 2017, 8: 2496-2506.
[77] , et al.. Pressure engineering of photovoltaic perovskites. Mater. Today, 2019, 27: 91-106.
[109] . Self-assembly of broadband white-light emitters. J. Am. Chem. Soc., 2014, 136: 1718-1721.
Article Outline
Mei Li, Tianbiao Liu, Yonggang Wang, Wenge Yang, Xujie Lü. Pressure responses of halide perovskites with various compositions, dimensionalities, and morphologies[J]. Matter and Radiation at Extremes, 2020, 5(1): 018201.