Doublet achromatic metalens for broadband optical retroreflector
Download: 914次
1. Introduction
Metasurfaces, artifically designed ultrathin two-dimensional (2D) materials composed of subwavelength resonators, exhibit extraordinary electromagenetic (EM) properties that are unattainable with natural materials. Metasurfaces have shown great potentials to tailor the wavefront of EM waves, which has led to various intriguing effects, such as light bending[1–
Initially, plasmonic metasurfaces/metagratings have been used to realize retroreflection, but the resultant experiment exhibits limited working bandwidth and/or a single incidence angle since the provided tangential momenta are fixed after the fabrication process[23–
2. Results and Discussions
2.1. Design principle
The transmissive achromatic gradient metasurface is schematically shown in Fig. 1(a), which enables a light source with a continuously changing wavelength to share the same focal length over a wide continuous range of incidence angles [Figs. 1(b) and 1(c)]. The required phase distributions of for an achromatic metalens should abide by[31,32]
![(a)–(c) Schematic of (a) a transmissive achromatic metasurface that enables a light source with a continuously changing wavelength to have (b), (c) the same focal point. (d)–(f) Schematic of (d) a reflective achromatic metasurface that bestows a twice tangential momentum to (e), (f) the incoming light source with continuously changed wavelength. (g)–(i) Schematic of a broadband retroreflector comprised of a transmissive achromatic metasurface combined with a reflective achromatic metasurface. (j), (k) Spectral phase profiles (left panel) for the central meta-unit and spatial phase profiles (right panel) along the radial direction for a (j) transmissive/(k) reflective achromatic metalens within an arbitrary wavelength range of λ∈[λmin, λmax].](/richHtml/col/2021/19/2/023601/img_001.jpg)
Fig. 1. (a)–(c) Schematic of (a) a transmissive achromatic metasurface that enables a light source with a continuously changing wavelength to have (b), (c) the same focal point. (d)–(f) Schematic of (d) a reflective achromatic metasurface that bestows a twice tangential momentum to (e), (f) the incoming light source with continuously changed wavelength. (g)–(i) Schematic of a broadband retroreflector comprised of a transmissive achromatic metasurface combined with a reflective achromatic metasurface. (j), (k) Spectral phase profiles (left panel) for the central meta-unit and spatial phase profiles (right panel) along the radial direction for a (j) transmissive/(k) reflective achromatic metalens within an arbitrary wavelength range of .
Equation (4) indicates that the required reflective metasurface is an achromatic metalens as well and has a focal length of F/2[29]. Figure 1(k) schematically depicts the required spectral phase profiles for the center meta-unit and spatial phase profiles along the radial direction for the achromatic metalens. A broadband retroreflector can thus be built with a combination of the transmissive and the reflective achromatic metalenses [Fig. 1(g)]. An incident light is anticipated to be reflected back to its incident direction over a continuous range of incidence angles, regardless of its wavelength [Figs. 1(h) and 1(i)].
2.2. Design and demonstration of broadband metasurface retroreflector
We introduce silicon metasurfaces with square pillars and square holes[31], as shown in Figs. 2(a) and 2(b), to provide diverse phase dispersions required to construct achromatic metalens. We firstly select the geometrical parameters for the center meta-unit of the transmissive (reflective) metalens with the phase of []. Afterwards, the meta-unit at an arbitrary radius [ () for the transmissive (reflective) metalens] can be selected out if its phase profile is mostly close to the distributions extracted by Eqs. (1) and (4). The optimized meta-units at () are selected out by use of the method of mean square error. Considering that there are multiple discrete wavelengths within the wavelength range [] and M meta-units for the transmissive (reflective) achromatic metalens, the mean square error is retrieved as () for the transmissive (reflective) achromatic metalens, where [] represents the phase profile of the th unit among all of the units. The meta-unit at () selected out as the mean square error is minimum.
![(a), (b) Schematics of (a) the transmissive meta-units comprised of silicon square pillars and square holes, and (b) the reflective meta-units comprised of silicon square pillars and square holes on a gold film. (c) Theoretical transmission phase profiles for the transmissive metalens at r0=6.3 μm (red dashed line), 4.5 μm (azure dashed line), and 0 (black dashed line), associated with the simulated transmission efficiencies (red, azure, and black dotted lines) and phase profiles (red, azure, and black solid lines) of three meta-units with WpL=0.18 μm, WhL=0.34 μm, and WhL=0.10 μm, respectively. (d) Theoretical reflection phase profiles for the reflective metalens at R0=5.4 μm (red dashed line), 3.6 μm (azure dashed line), and 0 (black dashed line), associated with the simulated reflection efficiencies (red, azure, and black dotted lines) and phase profiles (red, azure, and black solid lines) of three meta-units with WpR=0.20 μm, WhR=0.32 μm, and WhR=0.18 μm, respectively. The lattice constants along the x and y directions are P=0.45 μm, and the thickness of silicon is H1=0.6 μm. The silicon layer is covered by SU-8 polymer, with the thickness and refractive index being H2=2 μm and 1.555, respectively, and the refractive indices of other materials are extracted from Ref. [34].](/richHtml/col/2021/19/2/023601/img_002.jpg)
Fig. 2. (a), (b) Schematics of (a) the transmissive meta-units comprised of silicon square pillars and square holes, and (b) the reflective meta-units comprised of silicon square pillars and square holes on a gold film. (c) Theoretical transmission phase profiles for the transmissive metalens at r0 (red dashed line), (azure dashed line), and 0 (black dashed line), associated with the simulated transmission efficiencies (red, azure, and black dotted lines) and phase profiles (red, azure, and black solid lines) of three meta-units with , , and , respectively. (d) Theoretical reflection phase profiles for the reflective metalens at R0 (red dashed line), (azure dashed line), and 0 (black dashed line), associated with the simulated reflection efficiencies (red, azure, and black dotted lines) and phase profiles (red, azure, and black solid lines) of three meta-units with , , and , respectively. The lattice constants along the x and y directions are P , and the thickness of silicon is H1 . The silicon layer is covered by SU-8 polymer, with the thickness and refractive index being H2 and 1.555, respectively, and the refractive indices of other materials are extracted from Ref. [34].
Figures 2(c) and 2(d) show the transmission/reflection efficiencies and phase profiles of three transmissive/reflective meta-units, where the simulated phase agrees well with the theoretical value predicted by Eqs. (1) and (4). The numerical simulations are conducted by the finite-difference time-domain (FDTD) method with the commercial software Lumerical FDTD Solutions. It should be emphasized here that the used meta-units have four-fold symmetry that enables them to work with polarization independence. By arranging the meta-units along the directions to follow the achromatic phase profiles in Figs. 1(j) and 1(k), a broadband achromatic transmissive/reflective metalens with a continuous range of incidence angles can be successfully designed. Figure 3(a) shows that the achromatic transmissive metalens light waves of 1.35–1.95 µm with 0° and 16° incidence angles can be focused at the predesigned focal plane with a focal length of 22 µm. It should be noted here that light waves with other incidence angles between 0° and 16° within the wavelength range of interest can also be focused at the same focal plane, which has not been shown here. The simulated foci offsets and focal lengths are all consistent with the theoretically predicted values [Figs. 3(b) and 3(c)]. With the designed focal length of (11 µm) for the bottom reflective achromatic metalens, the simulated distributions of at the four wavelengths and the focal length versus wavelength are presented in Figs. 3(d) and 3(e), clearly indicating that all the incident light waves with normal incidence are reflected and focused at the same point. Achromatic reflective focusing performance can be verified using light illumination at an arbitrary wavelength within 1.35–1.95 µm as well.
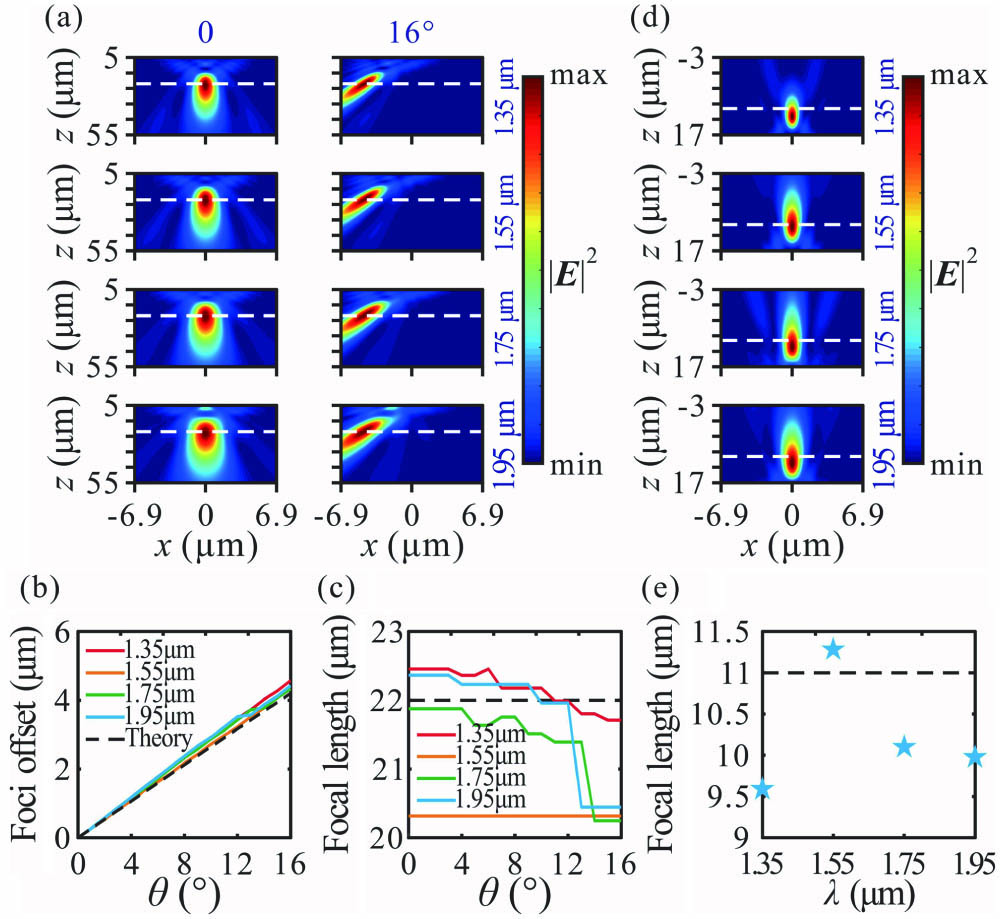
Fig. 3. (a) Distributions of E in the x–z plane for the transmissive metalens of 13.95 µm in diameter under the illumination of x-polarized light waves with 0° and 16° at 1.35, 1.55, 1.75, and 1.95 µm. (b) Foci offsets and (c) focal lengths versus incidence angle for four wavelengths (1.35, 1.55, 1.75, and 1.95 µm). (d) Distributions of E in the x–z plane for the reflective metalens of 12.15 µm in diameter under the normal illumination of x-polarized light waves at 1.35, 1.55, 1.75, and 1.95 µm. (e) Focal length of the reflective metalens versus light wavelength.
The broadband metasurface retroreflector can be built by placing the bottom achromatic reflective metalens at the focal plane of the transmissive one (Fig. 1). The dielectric spacer between the transmissive and reflective achromatic metalenses is assumed to be silica with the thickness being equal to . The electric-field distributions in the far-field region above the metasurface retroreflector can be retrieved by use of the inverse Fourier transform[35]:
![(a) Distributions of the real part of Eref,x for the broadband metasurface retroreflector under the illumination of x-polarized light waves for four wavelengths and three incidence angles (5°, 10°, and 15°). (b) In the upper semicircles, the normalized |Ax(φ,ky=0, θ, λ)|2 (solid lines, with respect to its maximum value) versus spatial angle φ and min[|rret(θ, λ)|2,|rnor(θ, λ)|2]/max[|rret(θ, λ)|2,|rnor(θ, λ)|2] (dotted arcs) under the x-polarized incidence for different wavelengths and incidence angles: 5° (red), 10° (orange), and 15° (green). In the lower semicircles, the normalized |ΔAx(φ,ky=0, θ, λ)|2 (dashed lines, with respect to its maximum value) versus spatial angle φ for different wavelengths and incidence angles: 5° (red), 10° (orange), and 15° (green).](/richHtml/col/2021/19/2/023601/img_004.jpg)
Fig. 4. (a) Distributions of the real part of Eref,x for the broadband metasurface retroreflector under the illumination of x-polarized light waves for four wavelengths and three incidence angles (5°, 10°, and 15°). (b) In the upper semicircles, the normalized (solid lines, with respect to its maximum value) versus spatial angle and (dotted arcs) under the x-polarized incidence for different wavelengths and incidence angles: 5° (red), 10° (orange), and 15° (green). In the lower semicircles, the normalized (dashed lines, with respect to its maximum value) versus spatial angle for different wavelengths and incidence angles: 5° (red), 10° (orange), and 15° (green).
The reflection angles versus the incidence angle within the wavelength range of interest are presented in Fig. 5(a) and are consistent with the predesigned retroreflection angles. The deviation of the reflection angle and the incidence angle is shown in Fig. 5(b), which clearly indicates that the difference is approximately 1°, almost within the entire wavelength range of interest. A small region merely exists in the left panel of Fig. 5(b), which is associated with the maximum difference of about 1.8° around 1.7 µm under -polarized incidence of 16°. The slight discrepancy between the simulated angles and the predesigned angles can be attributed to the fact that the phase dispersion of the used transmissive/reflective meta-units does not fully follow the conditions required by Eqs. (1) and (4). It should be noted here, as the reflective metalens has a limited size, there is a restriction for the maximum incidence angle. For an incidence angle of 16°, the focus of the transmissive metalens locates at around the boundary of the reflective metalens. For larger incidence angles, the performance of the broadband metasurface retroreflector will deteriorate, which has not been shown here. An effective method to increase the maximum incidence angle can be realized by use of a transmissive achromatic metalens with a smaller , which is at the sacrifice of the reduced radii of both metasurfaces.
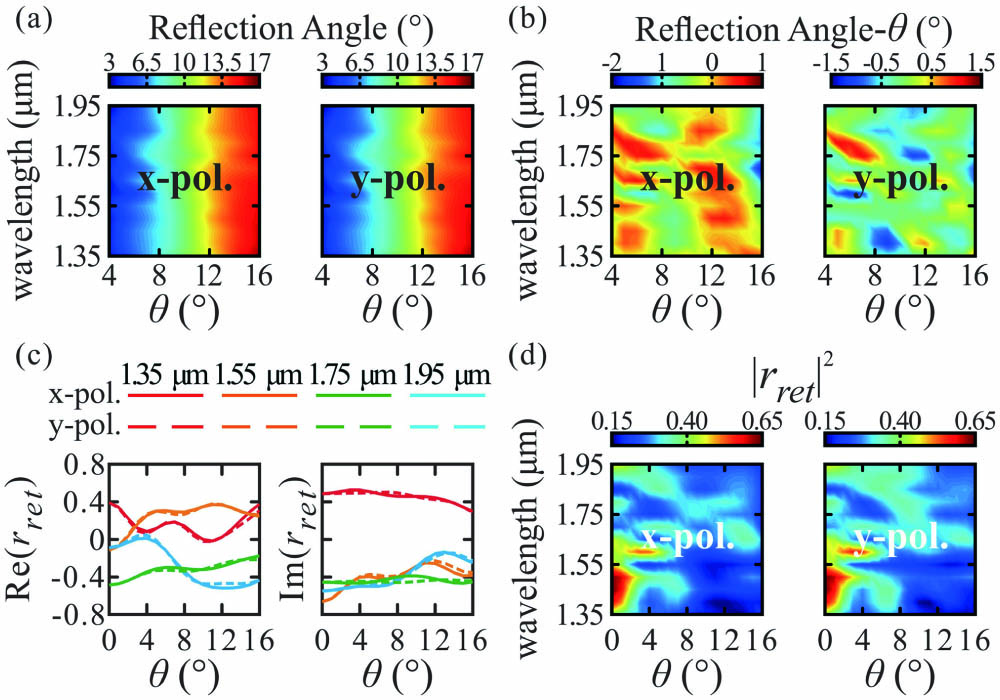
Fig. 5. (a) Reflection angles and (b) the difference between the reflection angles and the incidence angles versus incidence angle and wavelength under x- and y-polarized incidence. (c) Real and imaginary parts of the retroreflection coefficients rret versus incidence angles under x- and y-polarized incidence. (d) Values of rret versus incidence angle and wavelength under x- and y-polarized incidence.
As the used meta-units have four-fold symmetry and support a waveguide-like cavity resonance mode, the meta-units thus present gentle sensitivity to the polarization states of incidence light, even if the incident direction significantly deviates from the normal line[33]. Therefore, the resultant metasurface retroreflector is highly anticipated to operate with polarization independence. Other cross sectional shapes, such as a circle can also be an alternative for the meta-units. The retroreflection coefficients describing the relation between the incident plane wave component of incidence angle and its retroreflective counterpart for and polarizations can be estimated as
The under the -polarized incidence for different wavelengths and incidence angles are plotted in Fig. 4(b) and match well with the ratio between the two branches of the reflected light in the far-field region.
3. Conclusion
In conclusion, we have proposed a strategy of building broadband retroreflectors by use of transmissive and reflective achromatic metalenses. A silicon metasurface retroreflector at the telecom wavelengths is designed and numerically demonstrated by use of this design strategy, demonstrating the capability of retroreflecting polarization-independent light waves with the wavelength range between 1.35 and 1.95 µm over a continuous range of incidence angles from 0° to 16°. The design strategy can also be extended to the visible light band. The presented results point out a promising avenue to design complex metasurface photonic devices that are broadband and polarization friendly.
Article Outline
Ming Deng, Tangxuan Ren, Jian Wang, Lin Chen. Doublet achromatic metalens for broadband optical retroreflector[J]. Chinese Optics Letters, 2021, 19(2): 023601.