Time-resolved multiple imaging by detecting photons with changeable wavelengths
Download: 787次
Imaging is a general way of transporting information. For traditional imaging, a lens (or a set of lenses) is utilized to collect the photons passing through the object to a screen (or detector). Under a suitable geometric arrangement of the object, lens, and screen, a clear image will be obtained on the screen.
In 1995, as a new imaging method, ghost imaging, was demonstrated by using entangled two-photon pairs (signal and idler) created by spontaneous parametric downconversion (SPDC)[1]. Later, this imaging method was also theoretically and experimentally explored by using an unentangled light source[2
Recently, Lemos
In this work, we present and demonstrate an imaging method of detecting photons with variable wavelengths based on time-resolved correlation measurements. In our experiment, the wavelength of the photons detected by the detectors is different from that illuminating the objects. Since two nonlinear beta barium borate (BBO) crystals are used as the wavelength converters, the wavelength of the photons that are utilized to record images can be changed to match the sensitive range of the used detectors. Images of the objects have been reconstructed successfully by detecting the photons at wavelengths of 650, 810, and 1064 nm, respectively.
To describe our idea clearly, studying some properties of the time-resolved correlation of the laser pulses will be helpful. A femtosecond (fs) pulsed laser (Coherent, Inc.) as the light source has a central wavelength of 810 nm, a pulse duration of
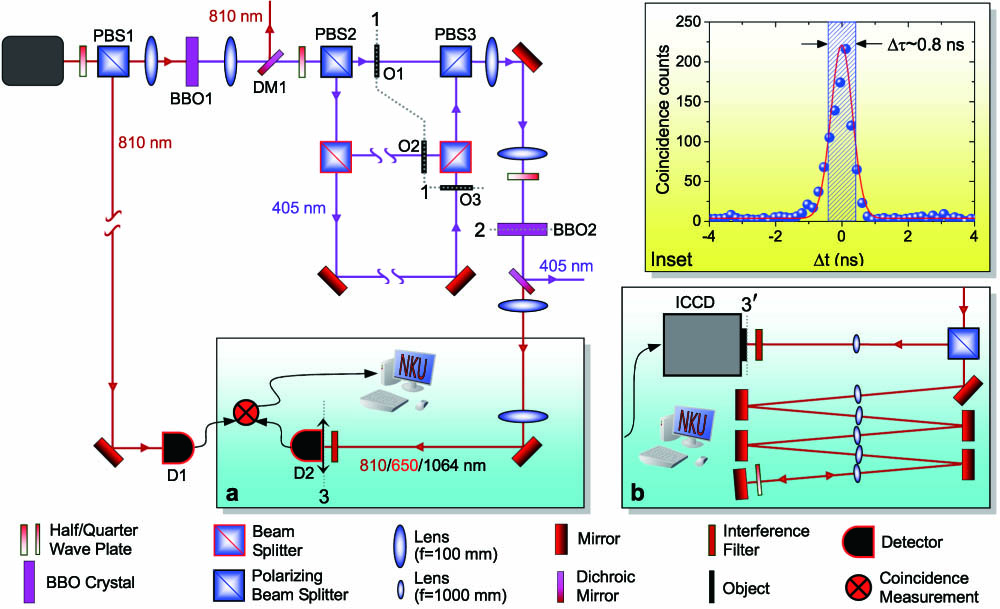
Fig. 1. Experimental setup for time-resolved multiple imaging with undetected photons. The light source is a fs pulsed light source at a central wavelength of 810 nm with a pulse duration of and a repetition rate of (the interval between two pulses is ). Two BBO crystals are used to realize the wavelength conversion. The process of second-harmonic generation happening in BBO1 will transform the wavelength from 810 to 405 nm. SPDC occurs in BBO2 and transforms the wavelength from 405 nm to the one that we need. DMs are used to remove the unwanted light. Here, DM1 transmits 405 nm light (96%) and reflects 810 nm light (97%). The other dichotic mirrors reflect 405 nm light (96%). There are three objects. O1 is letter “N”, O2 is letter “K”, and O3 is letter “U”. Plane “1” is the object plane; plane “2”, plane “3”, and plane “ ” are the image planes of the object. (a) The scanning imaging system arrangement, where the detector D2 needs to scan step by step to reconstruct the images of the objects. (b) The imaging with the ICCD and the image preserving delay compensation system arrangement. Insert: Rotation of BBO2 to generate the photons with the needed wavelength from SPDC. When , photons with a 1064 nm wavelength can be collected by D2. When , the wavelength of photons that we can collect is 650 nm. Inset: Measurement of the time-resolved correlation of the fs pulsed laser used, experimental data (blue symbols) and Gaussian fitting profile (red curve).
In our experiment below, we set the time window
Because there are three paths that can be chosen to propagate from PBS2 to PBS3 for photons, the time difference between the electrical signals output from D1 and D2 should have three possible values, corresponding to the three peaks contained in our experimental results [Fig.

Fig. 2. (Color online) Experimental results. (a) is an example of coincidence measurement between detector D1 and D2 for the scanning imaging system arrangement. (b1), (b2), and (b3) are images of the three objects reconstructed from the coincidence measurement. scanning steps are needed. Measurement time is about 5 seconds for each step. (b4) is a mixed image of the three objects achieved by extracting all information containing in the three peaks at each scanning step. (c1), (c2), and (c3) are images of the three objects by using the ICCD and image preserving delay compensation system arrangement. (c4) is the mixed image of the three objects recorded by triggering the ICCD with an internal pulse generator. (d) The red circles and the blue circles are the partial data of (b1) (along the red line) and (c1) (along the dark blue line), respectively, and the red and blue curves are the fitting results corresponding to the data shown by the red and blue circles.
As a comparison, we utilize another arrangement [Fig.
To further confirm our idea, we select the detected photons at two different wavelengths from 810 nm. We rotate BBO2 to change the phase-matching angle of BBO2. Thus, we can generate the photon pairs (idler and signal) at the different wavelengths we need. As mentioned above, when the 405 nm pump light is normally incident on BBO2, degenerate photon pairs at 810 nm will be generated. By rotating BBO2 with an angle of

Fig. 3. (Color online) Experimental results. (a1), (a2), and (a3) are images of the three objects reconstructed by detecting photons at 650 nm with the same dimension of scanning steps, and the measurement time is for each step. (b1), (b2), and (b3) are images of the three objects by using the ICCD arrangement at 650 nm with a dimension of corresponding to an area of . (c1), (c2), and (c3) are images of the three objects reconstructed by detecting photons at 1064 nm with the same dimension of scanning steps, and the measurement time is for each step.
In conclusion, by using the time-resolved property of the fs pulsed laser, correlation imaging by detecting photons with variable wavelengths are realized. Two nonlinear crystals are used to convert the wavelength of the photons. In our experiment, three objects (letters, “N”, “K”, and “U”) can be recorded at the same time. It should be noted that the wavelength of the photons illuminating the objects is different from that detected by the detectors during imaging, and the wavelength of the photons detected by the detectors can also be changed to match the sensitive range of the used detectors. In our current experiment, images of the objects are reconstructed clearly by detecting the photons at wavelengths of 650, 810, and 1064 nm, respectively. What is more, two schemes are used to achieve the multiple imaging. In the first scheme shown in Fig.
[1]
[2]
[3]
[4]
[5]
[6]
[7]
[8]
[9]
[10]
[11]
[12]
[13]
[14]
[15]
[16]
[17]
[18]
[19]
[20]
[21]
[22]
[23]
[24]
[25]
[26]
[27]
[28]
[29]
[30]
[31]
[32]
[33]
[34]
[35]
[36]
Lingjun Kong, Rui Liu, Yu Si, Zhouxiang Wang, Chenghou Tu, Yongnan Li, Huitian Wang. Time-resolved multiple imaging by detecting photons with changeable wavelengths[J]. Chinese Optics Letters, 2017, 15(8): 081101.