High-gain waveguide amplifiers in Si3N4 technology via double-layer monolithic integration
Download: 592次
1. INTRODUCTION
Integrated waveguide amplifiers and light sources are essential components for photonic integrated circuits that combine different functionalities on a single chip [1]. Currently, silicon-on-insulator [2,3], indium phosphide [4,5], and silicon nitride () [6,7] are three commercially available photonic platforms. The platform, due to its low propagation loss () and wide transparency window ( to 2.35 μm) [7], has achieved tremendous progress in passive applications including microwave photonics [8], nonlinear photonics [9], bio-sensing [10], quantum technology [11], and lidar [12]. The integration of active components such as amplifiers and lasers onto the passive platform will permit profiting from its excellent passive characteristics.
III-V semiconductor optical amplifiers have been hybrid integrated with the platform by butt-coupling to produce external cavity lasers [1315" target="_self" style="display: inline;">–
Recently, monolithic integration of rare-earth-ion-doped () aluminum oxide () and was demonstrated [18
In this work, we present an integrated high total gain (i.e., from passive waveguide to passive waveguide) optical amplifier in monolithically integrated onto the platform via a double-layer platform [38]. Different from previous integration methodologies in which the material was directly deposited onto elements [20,21,23,31] or sputtered into trenches within the platform [19,22], in our approach the and the waveguides are located in two individual layers separated by a thin film. The optical modes are therefore guided independently in each of the photonic layers permitting their independent optimization to minimize losses and to maximize the overlap between pump and signal modes on the active waveguides. The transfer of modes between the two layers is achieved via vertically tapered adiabatic couplers that exhibit low loss, broadband behavior, and high tolerance to overlay errors. Such tapers have already been demonstrated in our previous work [38,39]. A total gain as high as was achieved at 1532 nm for amplifiers with a 10 cm long gain section with more than 70 nm bandwidth of net gain.
2. RESULTS
Figure
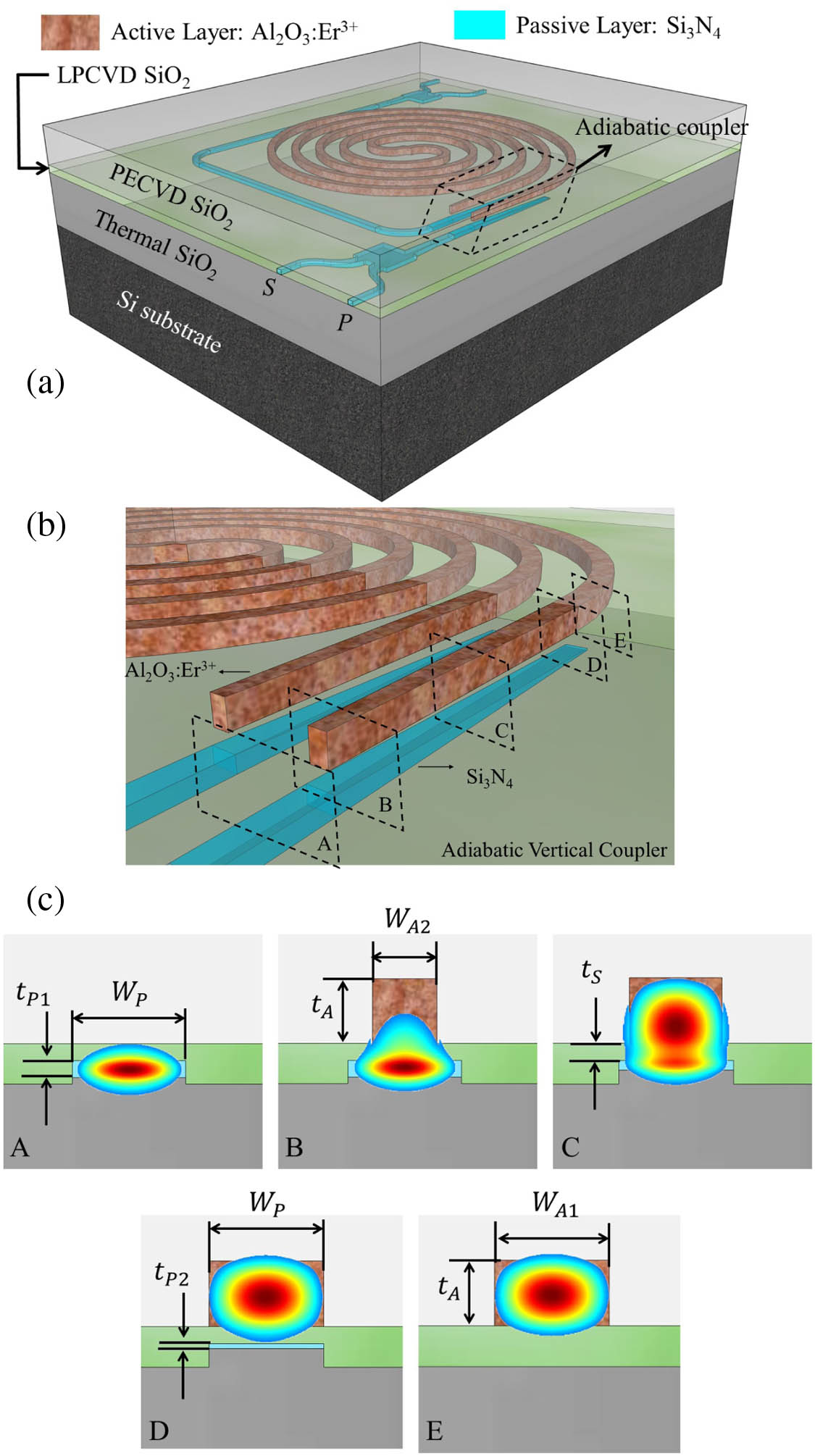
Fig. 1. Structure of the monolithically integrated amplifier. (a) 3D schematic of the whole amplifier chip with MMI-based multi/demultiplexer. P and S indicate the pump port and the signal port of the MMI multi/demultiplexer, respectively. (b) Schematic of the adiabatic vertical coupler, consisting of a vertical taper and a lateral taper. (c) Cross sections of the adiabatic coupler at different positions (A, B, C, D, E) indicated in (b) within the taper region along the propagation direction, and the related mode intensity profiles under transverse electric (TE) polarization. The different cross-sectional parameters are indicated. The field intensity is visualized in the rainbow color scale, where the highest intensity is represented by the red.
Between the and layers, a TEOS film was deposited as a spacer by LPCVD followed by an annealing step. The spacer provides not only a surface with good uniformity on top of which to deposit the layer but also protects the top surface of the waveguides during the fabrication of the fully etched waveguides. Simulations have shown that spacer thicknesses in the range 100–200 nm lead to both good optical coupling and good tolerance to the mask overlay errors [38] between the and waveguides in the adiabatic coupler. To achieve this spacer thickness, a chemical mechanical polishing step was applied to reduce the thickness of the as-deposited LPCVD TEOS layer from to [ in Fig.
Both the pump and the signal modes are transferred between the and waveguides through adiabatic couplers, shown in Fig.
For the active waveguide, monomode operation was chosen for both the pump (980 nm) and signal (C-band) wavelengths under transverse electric (TE) polarization. The overlap between the pump and signal modes was also maximized (88%) to achieve as high as possible optical gain. This value is higher than that under TM polarization. Furthermore, the on-chip multi/demultiplexers are also designed to operate under TE polarization. The total length of the spiral waveguide amplifier is designed to be 10 cm with a minimum radius of 250 μm, i.e., the radius of the S-bend in the center, at which the bend loss is less than and can be therefore neglected. The fully etched fabricated waveguides have a width of and a sidewall angle below 15° (Fig.
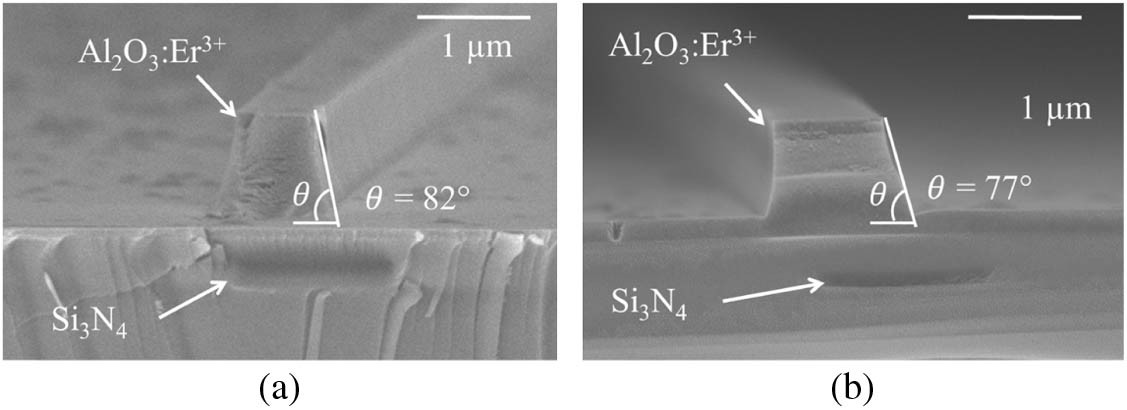
Fig. 2. SEM images of the cross sections (a) nearby the tip of the taper and (b) nearby the tip of the taper. The SEM images were captured from a cleaved sample from a monitor wafer with undoped on the platform.
The loss from input to output of waveguide consists of the loss of the two to adiabatic couplers () plus the total propagation loss of the spiral. The total loss per unit length of the waveguides, , consists of (i) the propagation loss (), mainly caused by scattering due to the roughness of the sidewalls of the waveguide, which is assumed to be uniform along the length of the waveguide, and (ii) the absorption loss (), due to the absorption of the ions excited from the ground state by the signal light. The absorption loss depends on the population of the ground state and, therefore, on the intensity of the signal used in the measurement. For the characterization of the gain of an amplifier using the signal enhancement method [23,36,41], the absorption loss in the small signal regime, i.e., for small enough signal so that the absorption cross section of the ions is constant, should be used. In this work, a non-destructive method [36,42] based on the measurement of the scattered light from the top of the waveguide was employed to measure the of the spirals. Top-view intensity images of the scattered signal light propagating along the integrated spiral amplifier, Fig.

Fig. 3. Characterization of losses. (a) Measured intensity of the light scattered along the length of the waveguide spirals at different wavelengths. Launched signal power is . (b) Measured absorption plus propagation losses of the waveguide spiral as a function of launched signal power.
Figure
Using the launched signal power of , was characterized in the full spectral window 1460–1640 nm, as shown in Fig. (a) Measured absorption plus propagation losses as a function wavelength for a launched signal power of
The coupler loss () was determined from the transmitted power of waveguides with different number of cascaded couplers fabricated in the same wafer as the spiral amplifiers. A mask overlay error below 0.4 μm was determined by means of reference Vernier rulers. The characterization was carried out at a wavelength of 1306 nm using the experimental setup described in our previous work [38,39], and it is plotted in Fig.
Furthermore, the insertion loss of the on-chip MMI multi/demultiplexer was experimentally characterized in the spectral window of 1460–1635 nm, as shown in Fig.
The net gain of the optical amplifiers (in decibels) from the input waveguide to the output waveguide was obtained using the following equation [23,36,42]: where the first term is known as signal enhancement, defined as the ratio of the output power of the signal with the pump on () corrected to eliminate the contribution of the amplified spontaneous emission (ASE) and the output power with the pump off (); the second term represents the total absorption and propagation losses of a spiral of length ; and the last term represents the losses introduced by the two adiabatic couplers. During gain measurements, both and are equally affected by the quality of the facets of the waveguides and the loss of the MMI multi/demultiplexers. Precise characterization of the loss of the couplers, the absorption loss, and the propagation loss is required to be carried out for the same amplifier. This avoids introducing the variations of between devices located at different areas of the wafer where the sputtered ion concentration slightly varies. This leads to more accurate and reliable gain values (shown below in Fig.
Figure
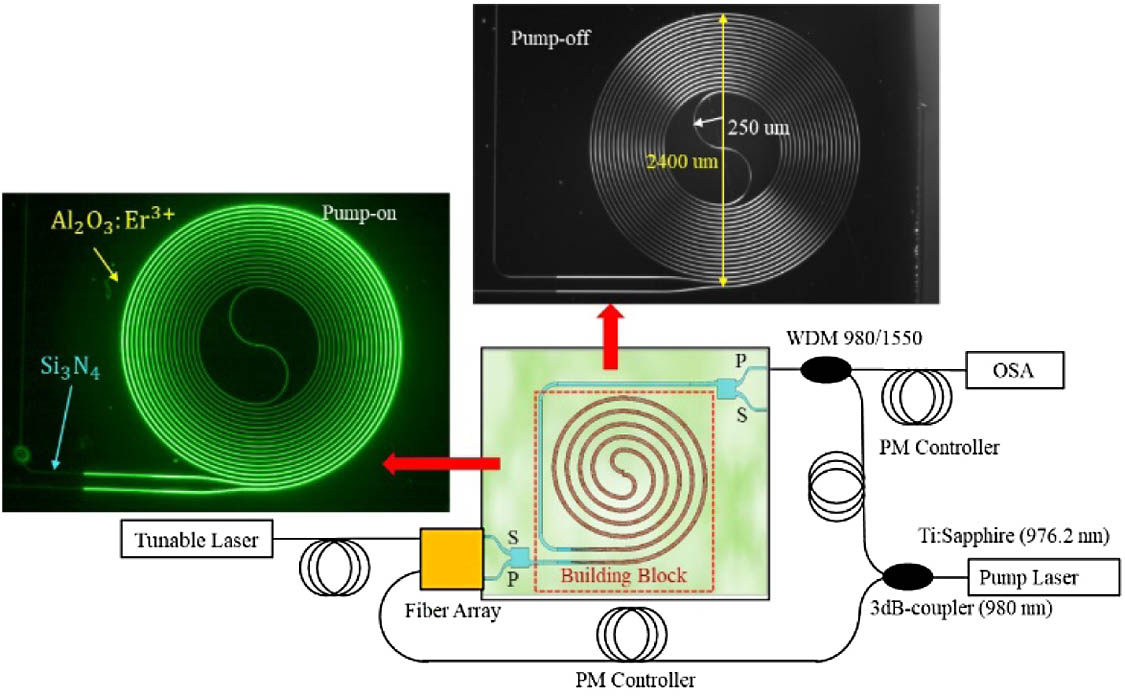
Fig. 5. Experimental setup utilized for the measurement of the net gain on the integrated spiral amplifier. The pump light, at 976.2 nm, is split by a 3 dB coupler (operating wavelength 980 nm) and injected into the amplifier chip from both the input and output sides via MMI multi/demultiplexers. The signal, from a tunable laser operating in the C-band, is injected from the left and collected on the right side and directed to the optical spectrum analyzer. The photographs represent the integrated spiral amplifier in the pump-on and pump-off cases. The pitch between adjacent loops of the spiral is 40 μm.
The ASE was subtracted from the signal spectra prior to the calculation of the signal enhancement using a similar method presented in Ref. [23]. The transmission spectrum collected by the OSA under the pump-off is . When the pump is on, the spectrum of the amplified signal power () includes the ASE power which equals to the background power from the spectral curves (). For instance, Figs.
Figure
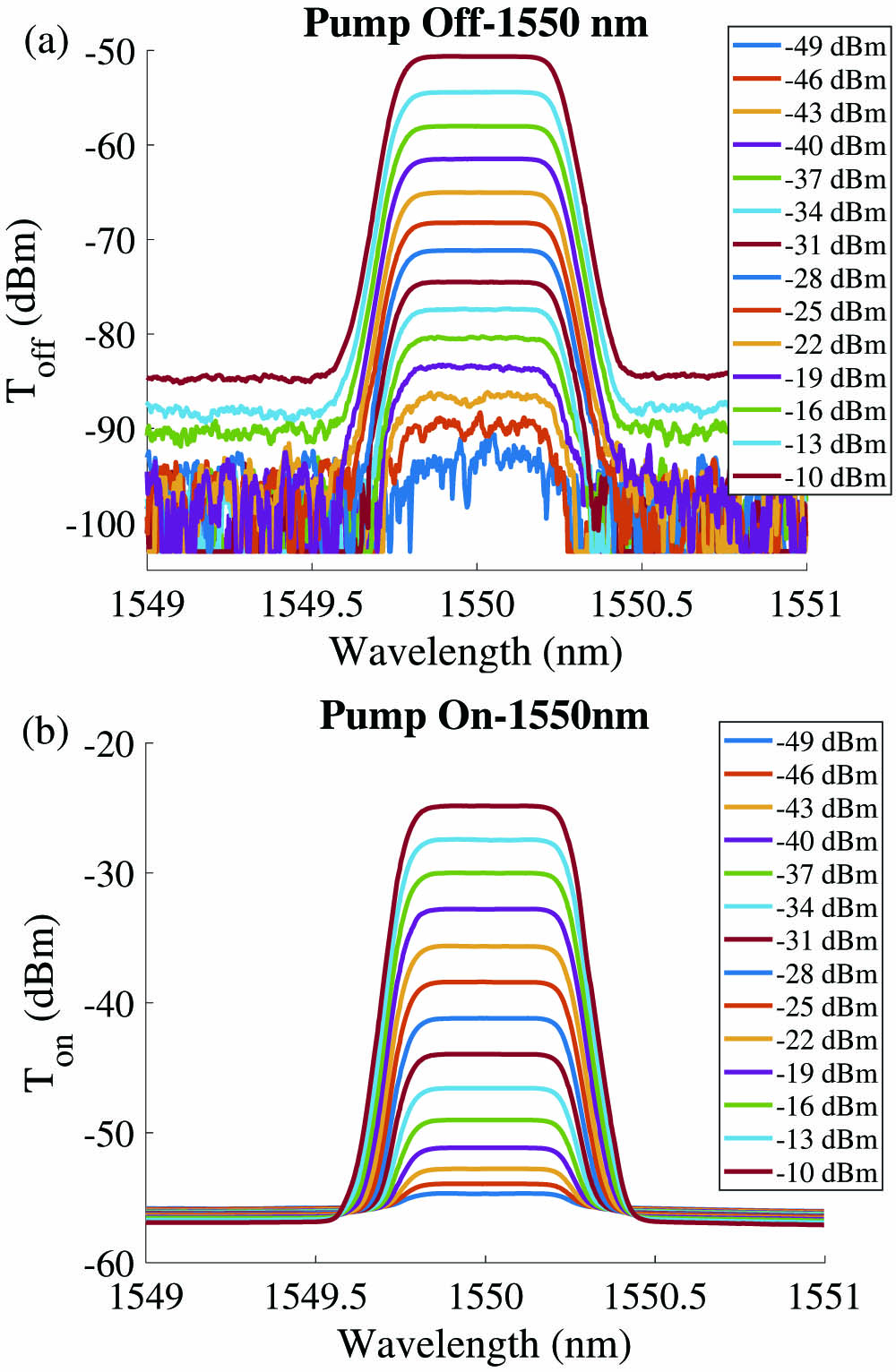
Fig. 6. Measured spectra from the OSA at the wavelength of 1550 nm under (a) pump-off case and (b) pump-on case. The legend indicates the launched signal powers. The launched pump power is .

Fig. 7. Net gain measurements. (a) Net gain of the integrated amplifier as a function of launched pump power for different launched signal powers (1532 nm of wavelength). (b) Net gain as a function of launched signal power for three different wavelengths within the C-band. The launched pump power is 50 mW. The dashed lines indicate 3 dB drop from the maximum gains for the three wavelengths. (c) Net gain as a function of wavelength for a launched signal power of 30 dBm and launched pump power of 50 mW. A bidirectional pumping scheme is applied. The reported launched power corresponds to the sum of the powers launched in each of the two input ports.
3. CONCLUSION
As a summary, we demonstrated an amplifier monolithically integrated in technology. By using a simple double-layer photonic platform, active photonics are enabled in the platform via a scalable wafer-scale process. A net gain of was obtained for a spiral length of 10 cm. The results are comparable to the highest net gain in a non-integrated amplifier from previous work in our group [36]. A broad bandwidth net gain operation over 70 nm wavelength was also demonstrated. These results show the potential for monolithic integration of high optical gain in photonics, empowering applications such as data transmission, optical computing, quantum technology, and lidar chips for autonomous driving applications, for which on-chip optical gain is required.
4 Acknowledgment
Acknowledgment. The authors would like to thank LioniX International, Michiel de Goede, and Sergio Vázquez-Córdova for useful supports and discussions.
Article Outline
Jinfeng Mu, Meindert Dijkstra, Jeroen Korterik, Herman Offerhaus, Sonia M. García-Blanco. High-gain waveguide amplifiers in Si3N4 technology via double-layer monolithic integration[J]. Photonics Research, 2020, 8(10): 10001634.