Upconversion-luminescent hydrogel optical probe for in situ dopamine monitoring
Download: 706次
1. INTRODUCTION
Dopamine (DA) is a central monoamine neurotransmitter involved in the regulation of a wide range of complex processes, including normal brain function, emotions, muscle movement, and hormones [1–3]. High levels of DA in the brain are responsible for reward and pleasurable feelings, whereas its deficiency can lead to stress, depression, and motor disorders. Impaired DA transmission is associated with many neurological diseases, including epilepsy, drug addiction, memory loss, schizophrenia, Parkinson’s disease, attention deficit hyperactivity disorder, and psychiatric problems [4,5]. In addition, recent studies have shown that DA concentrations in tumor tissues are usually lower than those in normal tissues [6]. Therefore, the development of efficient approaches for quantitative measurement of DA should facilitate diagnosis and treatment of neurodegenerative disorders and cancers in clinics.
To date, several methods have been developed for DA detection, including microdialysis [7], liquid chromatography (HPLC) [8], electrochemistry [9–13], colorimetry, and luminescent spectroscopy techniques [14–20]. Microdialysis and HPLC have long been the gold standard for quantitative measurement of DA but suffer from the limitations of complicated instrumentation and high cost. Fast-scan cyclic voltammetry (FSCV), one of the most popular electrochemical methods, has been successfully utilized to quantify DA with high temporal resolution and sensitivity that, however, has difficulties in distinguishing DA from other competing species of similar oxidation potential. Despite the recent progress achieved in improving the selectivity of electrochemical methods, they are still limited to a few electroactive species [21]. Luminescent and colorimetric probes such as upconversion nanoparticles (UCNPs) and quantum dots (QDs) have also shown great potential in detecting DA because of their high sensitivity and selectivity [22–25]. However, these methods require the nanoprobes to be dispersed in the analyte sample for the subsequent fluorescence analysis, which suffers from sample contamination and is susceptible to environmental interference. An attractive alternative for the design of DA sensing probes is to use functionalized optical fibers due to their miniaturized size, low cost, remote sensing capability, and electromagnetic interference (EMI) immunity [26–30]. For example, Agrawal et al. demonstrated an optical fiber probe functionalized with silver nanoparticles and polyethylene glycol (PEG) for DA detection based on the localized surface plasmon resonance (LSPR) effect [26]. Kim et al. proposed a miniaturized and wireless optical neurotransmitter sensor by coating an optical fiber tip with modified QDs for real-time DA sensing [29]. However, optical fiber sensors using conventional optical materials such as silica and plastics are generally rigid and fragile, making them incompatible with the soft and elastic biological tissues [31–33]. To address these problems, soft and implantable optical waveguides made from polymer hydrogels such as polyacrylamide (PAAm), poly-(ethylene glycol) diacrylate (PEGDA), and agarose, have been developed with favorable optical and physio-mechanical properties for light delivery in deep tissues [34–38]. In particular, there have also been efforts in integrating photonic functions into hydrogel waveguides for biosensing (e.g., glucose monitoring [37], metal ion sensing [38,39], and toxicity detection [40]) and photomedicines [41–43]. It is highly desirable to fabricate a hydrogel optical fiber (HOF) platform that is capable of quantitative and selective monitoring of DA in situ while integrating high softness and biocompatibility.
Herein, we report a soft and biocompatible HOF sensor utilizing lanthanide ()-doped UCNPs for quantitative and selective detection of DA. The UCNPs synthesized with a core of and a shell of () are immobilized in the HOF through precursor doping. A DA molecule consists of two hydroxyl groups and one amino group, and its spontaneous oxidation produces DA quinone species (ox-DA). The ox-DA has a broad absorption peak, which overlaps with the emission peak of the UCNPs. Under near-infrared (NIR) excitation, the UCNPs generate 450 nm emissions, and the luminescence intensity is selectively quenched by DA based on luminescence energy transfer (LET) between them [23]. The HOF, capable of efficient light guiding, is made from transparent biocompatible PEGDA hydrogels, which enables facile excitation and emission collection of the UCNPs as well as endows the sensor with high mechanical compliance and biosafety. Systematic characterizations are performed, where the UCNPs-incorporated HOF (UCNPs-HOF) sensor shows a linear response to DA over the range of 0–200 μM (1 M = 1 mol/L) with detection limit as low as 83.6 nM. Furthermore, the UCNPs doped in HOF exhibit a high photostability even when immersed in aqueous samples of various pH values and temperatures, suggesting their high robustness to environmental disturbances. We show that the hydrogel optical sensor could be used as a point-of-care sensing probe for quantitative and in situ monitoring of DA, which could find useful applications in clinical analysis and diagnosis of DA-associated diseases.
2. EXPERIMENTAL SECTION
2.1 A. Fabrication of the UCNPs-HOF Sensor
The UCNPs are synthesized via the solvothermal method [44] and the obtained UCNPs, dispersed in deionized (DI) water, are used for sensor fabrication. The sensor is made of a PEGDA hydrogel fiber doped with UCNPs. The UCNPs/PEGDA hybrid precursors are prepared by mixing UCNPs (0.1% w/v) with degassed solutions of 40% w/v PEGDA (700 Da, Sigma-Aldrich) and 1% w/v 2-hydroxy-2-methyl-propiophenone (Sigma-Aldrich) in DI water. The UCNPs-HOF is fabricated by injecting the prepared precursor into a polyethylene tube mold (inner diameter, 1 mm) through a syringe and curing by ultraviolet (UV) exposure (365 nm, ) for 5 min. For laser excitation and emission collection, a silica multimode fiber (MMF) (core/cladding, 200/215 μm) is coupled to the UCNPs-HOF by inserting the MMF tip into the center of the precursor-containing mold prior to UV curing. Afterwards, the UCNPs-HOF pigtailed with MMF is extracted out of the mold by water pressure.
2.2 B. Equipment and Characterization
The microscopic images of the UCNPs are taken by a 120 kV transmission electron microscope (Tecnai Spirit). The upconversion luminescence (UCL) emissions of the UCNPs under 980 excitation are measured by a CCD spectrometer (Thorlabs, CCS100), which has a scanning wavelength range of 350–700 nm, fully covering the UCL spectrum. Energy-dispersive X-ray (EDX) analysis of the UCNP samples is performed with an energy-dispersive spectrometer (Oxford Instruments). Statistical size distribution of the UCNPs is obtained from 200 UCNPs. Absorption spectroscopy is carried out with a UV-Vis spectrophotometer (Agilent 8453) to measure the absorption spectra of UCNPs-PEGDA hydrogels and DA samples. Temperature dependence of the UCNPs-HOF sensor is investigated by using a heating water bath equipped with a thermocouple (resolution, 0.1°C) for temperature calibration. For pH characterization, Tris-HCl (Sigma-Aldrich) and Tris base (Sigma-Aldrich) are used to prepare pH buffer solutions () with pH ranging from 4.5 to 10.5.
2.3 C. Experimental Setup for DA Detection
A fiber-coupled laser diode at 980 nm is employed to interrogate the UCNPs-HOF sensor through a 50:50 fiber coupler and MMF. The excited UCL emission is collected and guided to a portable spectrometer (Thorlabs, CCS100) for spectral analysis. A short-pass optical filter with cut-off wavelength of 850 nm is utilized to remove the residual excitation light in front of the spectrometer. Tris-HCl buffer solution (, ionic ) is used to prepare DA samples with concentrations ranging from 0 to 200 μM. For DA sensing, the UCNPs-HOF sensor is immersed in aqueous DA samples, and the corresponding changes in UCL spectra are continuously recorded by the spectrometer.
3. RESULTS AND DISCUSSION
DA-sensitive lanthanide ()-doped UCNPs, capable of converting NIR radiation into short-wavelength visible emissions, are synthesized as the sensing components. The UCNPs are made up of an active core of and an inert shell of . Transmission electron microscopy (TEM) observation of the UCNPs shows uniform and monodisperse particles [shown in Fig. 1(a)]. The size distribution of the UCNPs taken from the TEM is fitted by a Gaussian function, indicating an estimated size of 37.86 nm [shown in Fig. 1(b)]. The composition of the UCNPs is analyzed by energy-dispersive X-ray analysis (EDXA), which confirms the elemental existence of Na, Y, F, Tm, and Yb [shown in Fig. 1(c)]. Figure 1(d) shows the schematic diagram of the upconversion process of the . While the core of provides visible emissions under 980 nm excitations via energy transfer from the ions to ions, the inert shell of serves as a protecting layer that improves the UCL [45]. Figure 1(e) shows the UCL spectra of the aqueous UCNPs sample, where three distinct emission bands centered at 450, 475, and 645 nm are observed, corresponding to the , , and transitions of , respectively [23]. Whenthe excitation power is increased from 40 to 120 mW, a linear increase in the peak emission intensities (@ 450 nm) of UCNPs is observed.
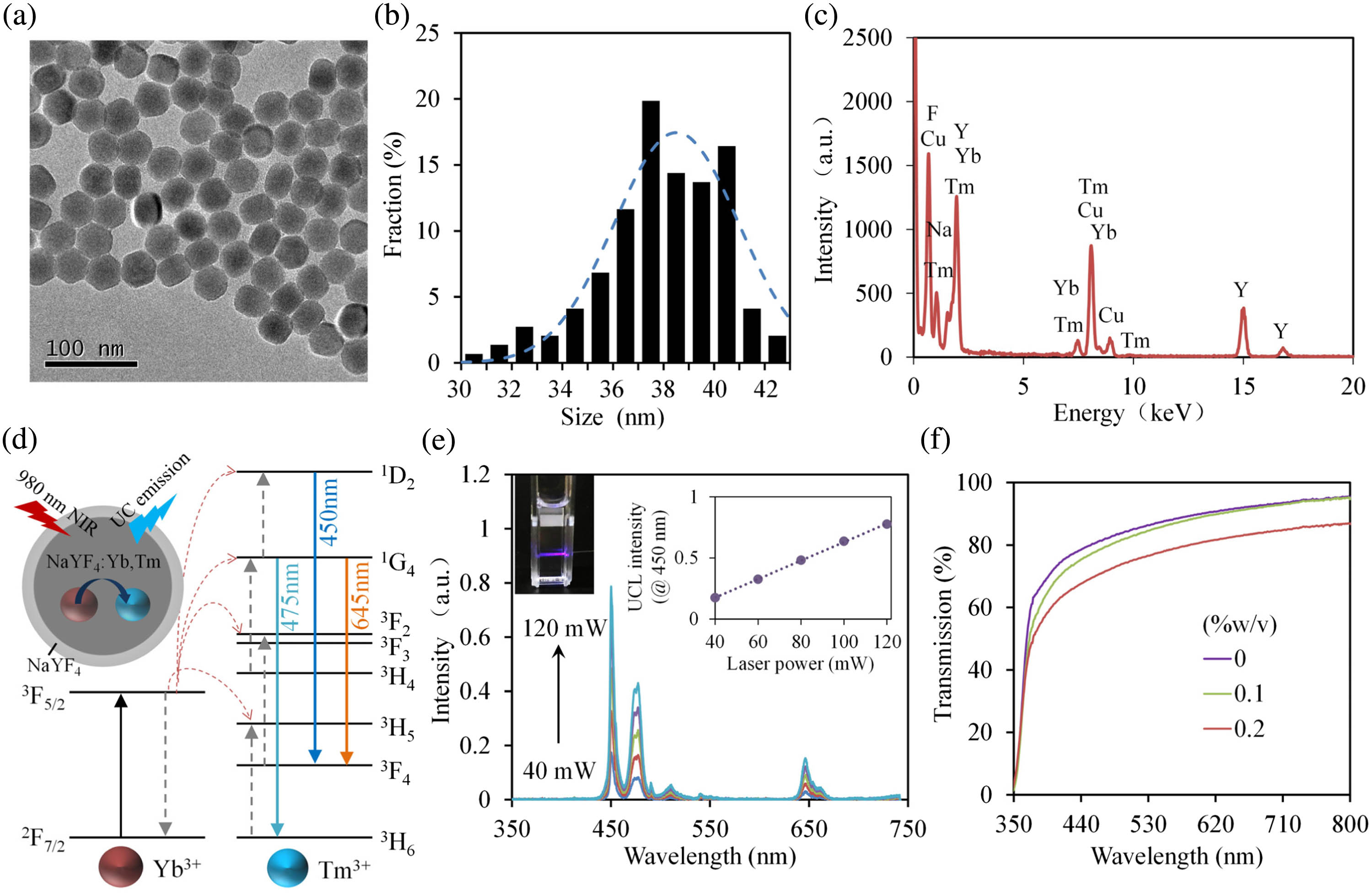
Fig. 1. (a) TEM images, (b) size distribution, (c) EDXA, and (d) schematics and upconversion process of the UCNPs. (e) Emission spectra of the UCNPs dispersed in water under different excitation powers. The concentration of the UCNPs is set at 0.1% w/v. The inset graph shows a linear relationship between emission intensity and excitation laser power. (f) Transmission spectra of hydrogel incorporated with various concentrations of UCNPs.
The synthesized UCNPs are assembled into biocompatible PEGDA hydrogels to fabricate the HOF sensor through precursor doping and UV-induced polymerization. PEGDA hydrogels are soft and biocompatible polymers that are widely used in biosensing and biomedicine [46,47]. In addition to their excellent physio-mechanical properties, PEGDA hydrogels are highly transparent in a broad spectral range, favorable for optical sensing. The pore size of 700 Da PEGDA hydrogels is less than 2 nm, and thus the large-size UCNPs could be effectively immobilized within the hydrogel matrix through physical entrapping. Absorption spectroscopy is employed to investigate the effect of the doping UCNPs on the optical transparency of the hydrogels [shown in Fig. 1(f)]. The UCNPs/PEGDA hydrogels show decreased transparency with the increasing concentrations of UCNPs, ascribed to the increased light scattering and absorption. As the doping concentration increases to 0.2% w/v, the light transmission at 450 nm decreases to nearly 60%. To achieve high light-guiding efficiency, we choose 0.1% w/v UCNPs to fabricate the UCNPs-HOF in the following experiments.
A simple molding process is employed to fabricate the UCNPs-HOF from the hybrid precursors of UCNPs and PEGDA, as depicted in Fig. 2(a). For light coupling, a silica multimode fiber (MMF) is inserted into the tube mold and aligned to its central axis prior to UV curing. To confirm its light-guiding capability, green laser light at 532 nm is launched into the UCNPs-HOF [shown in Fig. 2(b)]. The UCNPs-HOF can effectively guide light even when tied into knots, demonstrating excellent optical performance and mechanical flexibility [shown in Fig. 2(c)]. When illuminated by NIR laser at 980 nm, blue UCL emission is observed along the UCNPs-HOF as a result of the upconversion process in the incorporated UCNPs [shown in Fig. 2(d)].

Fig. 2. (a) Fabrication of the UCNPs-HOF by molding and UV-induced crosslinking. (b) Coupling of a 532 nm laser to the UCNPs-HOF. (c) Mechanical flexibility. The UCNPs-HOF can effectively guide light even when tied into a knot. (d) Photograph showing blue UCL emission of the UCNPs-HOF under the illumination of an excitation laser at 980 nm. (e) Optical setup for interrogation of the sensing UCNPs-HOF. (f) Long-term stability.
Using the UCNPs-HOF as a DA sensing probe, we develop a compact optical setup for the sensor interrogation, as shown in Fig. 2(e). A 980 nm laser () is launched into the sensing fiber through a 50:50 fiber coupler and MMF, and the UCL emission is guided to a spectrometer for spectral analysis. The UCNPs-HOF is immersed in aqueous samples at room temperature, during which its emission spectrum is continuously recorded. To confirm the immobilization of UCNPs in the HOF, the UCL emission spectrum is continuously monitored for 48 h [shown in Fig. 2(f)]. Negligible changes are observed in the UCL intensities, suggesting excellent photo-stability and no leakage of UCNPs from the hydrogel matrix.
For implantable sensing applications, temperature and pH are important factors to be considered since body temperature highly fluctuates over time, and the pH values of various biological samples (such as blood, urine, or cerebrospinal fluid) may differ greatly. For accurate sensing, it is essential to develop a DA sensor that is insensitive to changes of temperature and pH. As such, we investigate the dependence of the UCL intensities of the UCNPs on pH and temperature. Figure 3(a) shows the UCL spectra of the sensor when immersed in a Tris-HCl buffer solution (, ) at various temperatures. The UCL intensity maintains good stability, with a maximum fluctuation of 6.5% in the temperature range of 29–52°C, fairly large to cover the variation of normal body temperature [shown in Fig. 3(b)]. Figure 3(c) shows the UCL intensities of the sensing fiber in buffer solutions with pH ranging from 4.6 to 10.6, demonstrating that the UCL intensity is insensitive to the changes of pH.
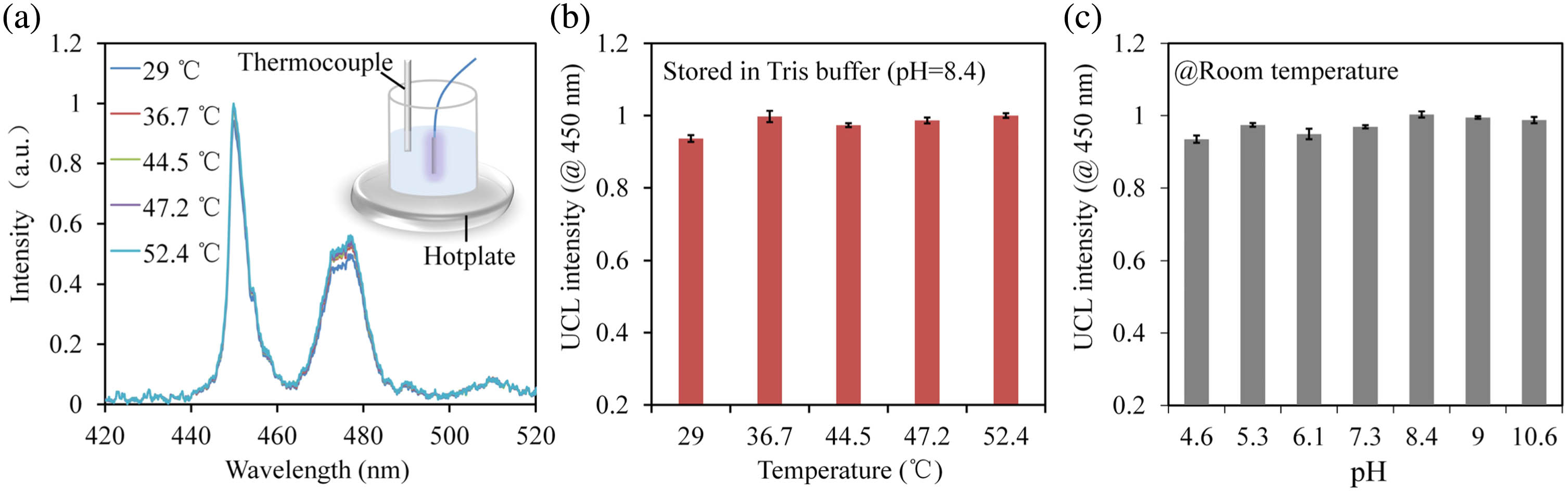
Fig. 3. (a) Dependence of the UCL spectrum on temperature. The inset image describes the corresponding experimental setup, where the sensor is immersed in a heating water bath (Tris-HCl buffer, , ) and a thermocouple is employed for temperature calibration. (b) UCL intensities at 450 nm under different temperatures (Tris-HCl buffer, , ). (c) UCL intensities at 450 nm under different pH values at room temperature.
The mechanism of the UCNPs-HOF sensor for DA sensing is based on the quenching effect of the UCNPs towards DA [shown in Fig. 4(a)]. The design of HOF enables not only fast exchanges with the surrounding analytes but also efficient light confinement for high signal-to-noise ratio (SNR) [38]. The oxidation of DA molecules yields ox-DA, which has a broad absorption peak overlapping the emission peak of UCNPs around 450 nm [shown in Fig. 4(b)]. Thus, the energy created by NIR excitation of the donor UCNPs is reabsorbed by the acceptor ox-DA, resulting in UCL quenching [23]. Selectivity of a specific biosensor is crucial for practical applications due to potential presences of other interfering species. To investigate the selectivity of the UCNPs-HOF sensor to DA, the sensor is tested with aqueous samples separately containing DA, , KCl, NaCl, glycine (Gly), L-glutamate (L-Glu), glucose (GLU), uric acid (UA), ascorbic acid (AA), and S-adenosylmetionine (SAM), which are commonly present in human biofluids [shown in Fig. 4(c)]. The quenching ratios () of the UCNPs-HOF sensor towards DA samples are much higher than those of other samples, which are close to 1 [shown in Fig. 4(d)]. The high selectivity of the UCNPs-HOF sensor for DA detection is attributed to the specific emission–reabsorption mechanism, making it robust against potential coexistence of other species.
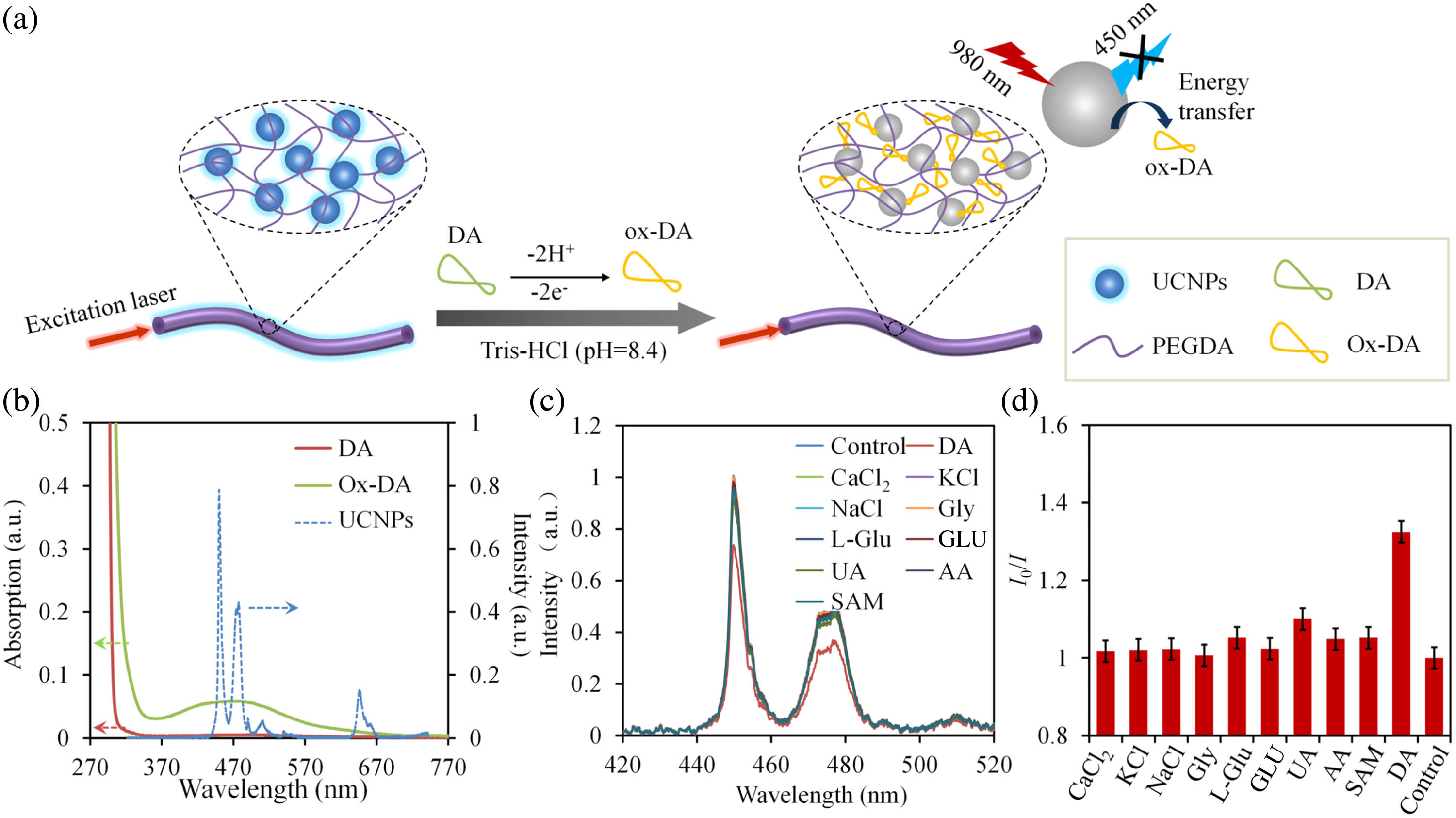
Fig. 4. (a) Mechanism of the UCNPs-HOF for DA sensing. (b) Absorption spectra of DA and ox-DA, and emission spectrum of the UCNPs-HOF. (c) Emission spectra of UCNPs-HOF immersed in different samples containing DA, , KCl, NaCl, glycine (Gly), L-glutamate (L-Glu), glucose (GLU), uric acid (UA), ascorbic acid (AA), and S-adenosylmetionine (SAM). The concentration of each sample is kept constant at 100 μM. (d) Selectivity of the UCNPs-HOF sensor.
As we have described in Fig. 3(c), the UCL intensity of UCNPs-HOF is insensitive to the changes of pH. It must be noted, however, that the spontaneous oxidation process of DA is highly pH-dependent. The oxidation process of DA in alkaline environment is more facile than that in acidic environment, resulting in higher efficiency of the UCL quenching at stronger alkaline pH [shown in Fig. 5(a)]. Therefore, for biological samples at different pH, calibration is required before DA testing. Figure 5(b) shows the response of the UCNPs-HOF sensor to various concentrations of DA. The quenching ratio of the sensor shows a linear relationship with the increasing concentration of DA in a range of 0–200 μM, demonstrating its capability in quantitative measurements [shown in Fig. 5(c)]. The quenching behaviors of the UCNPs-HOF sensor towards DA can be described in the form of the Stern–Volmer equation [22]:
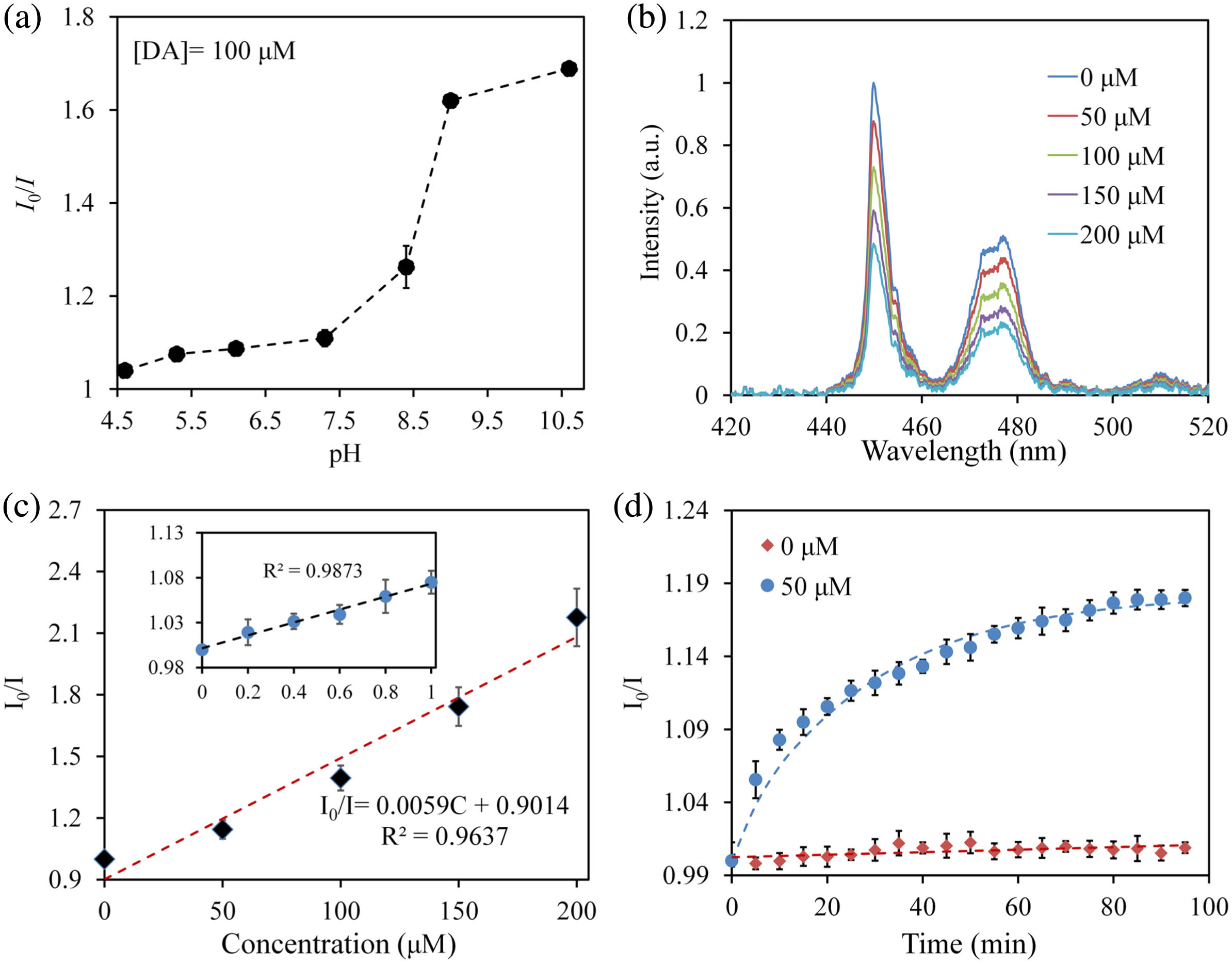
Fig. 5. (a) UCL quenching ratios of the UCNPs-HOF for DA sensing at different pH values. The DA concentration is set at 100 μM. (b) Emission spectra of the UCNPs-HOF versus the concentration of DA (Tris-HCl buffer, , ). (c) Corresponding calibration curve of the UCNPs-HOF for DA detection in the range of 0–200 μM. Inset shows a linear plot in a small range of 0–1 μM. (d) Time response of the UCNPs-HOF sensor.
Table 1. Determination of DA in Samples of Human Blood Serum
|
4. CONCLUSIONS
In summary, we have developed a soft and biocompatible optical DA sensor based on UCNPs-doped HOF. The UCNPs were synthesized with a core of and a shell of , which could generate visible UCL emissions at 450 nm upon NIR excitation. The HOF provided an implantable, biocompatible, and versatile platform for excitation and emission collection of the UCNPs, as well as analyte exchanging with the surrounding environments. DA molecules were quantified from UCL intensities at 450 nm through LET between UCNPs and oxidation products of DA. We showed that the UCNPs-HOF sensor was capable of accurately detecting DA in the range of 0–200 μM with high linearity, selectivity, and sensitivity (LOD of 83.6 nM). The soft and biocompatible UCNPs-HOF sensor is expected to offer a promising point-of-care diagnostic tool for quantitative and in situ monitoring of DA in clinics.
5 Acknowledgment
Acknowledgment. J. G. acknowledges funding from the National Natural Science Foundation of China (No. 61805126) and the Postdoctoral Innovation Talents Support Program. L. K. acknowledges the support from Tsinghua University Initiative Scientific Research Program (No. 20193080076).
L. K. and J. G. conceived the idea. B. Z. performed the experiments. J. G., B. Z., and L. K. analyzed the data. All authors contributed to the editing of the manuscript.
Article Outline
Bingqian Zhou, Jingjing Guo, Changxi Yang, Lingjie Kong. Upconversion-luminescent hydrogel optical probe for in situ dopamine monitoring[J]. Photonics Research, 2020, 8(11): 11001800.