Experiments on the characteristics of underwater electrical wire explosions for reservoir stimulation
1 I. INTRODUCTION
Electrical explosion of a metal wire driven by a pulsed current is a common method for generating (i) a plasma with relatively high temperature and density, (ii) pulsed electromagnetic radiation, and (iii) shock waves (SWs), and this method is used widely in the fields of Z-pinch plasma confinement, warm dense matter, nano-powder preparation, and reservoir stimulation, among others.
Naturally, different SW parameters are required for different applications. By changing the stored energy, circuit parameters, or wire load, SWs with different parameters can be obtained. For a specific circuit with fixed stored energy, in most cases there is an optimal mode with the largest energy deposition and strongest SW generation.
Since 2010, with the aim of developing the technology of controllable SWs based on underwater pulsed discharge for reservoir stimulation (coalbed methane, shale gas, etc.), a series of investigations has been conducted at Xi’an Jiaotong University led by Professors Aici Qiu and Yongmin Zhang. To address the growing demands from practical engineering, three generations of repetitive SW sources have been proposed and put into practice in the past few years, with the maximum SW energy of a single shot reaching more than 8 kJ.
In this review, we present the main results of our recent work on microsecond wire explosions for reservoir stimulation. We describe the technical details of an experimental setup that we established for researching microsecond wire explosions. On this platform, various wire loads have been exploded by different pulsed currents, and we have detected and analyzed the main processes, such as SWs and optical emission. We also summarize how the parameters of (i) the pulsed power source, (ii) the wire, and (iii) the medium influence the wire-explosion characteristics, and we present general principles and regulation methods for discharge type, energy deposition, SWs, and optical radiation. This review is intended to provide a better understanding of the characteristics of UEWE and to act as a reference for engineering aimed at producing underwater SWs in wells or oceans.
The rest of this is review is arranged as follows. In Sec.
2 II. EXPERIMENTAL SETUP AND DIAGNOSTICS
2.1 A. Platform for exploding a wire under water
An experimental setup (Single Wire Explosion platform 2, abbreviated as SWE-2) designed for microsecond wire explosions was established at Xi’an Jiaotong University.
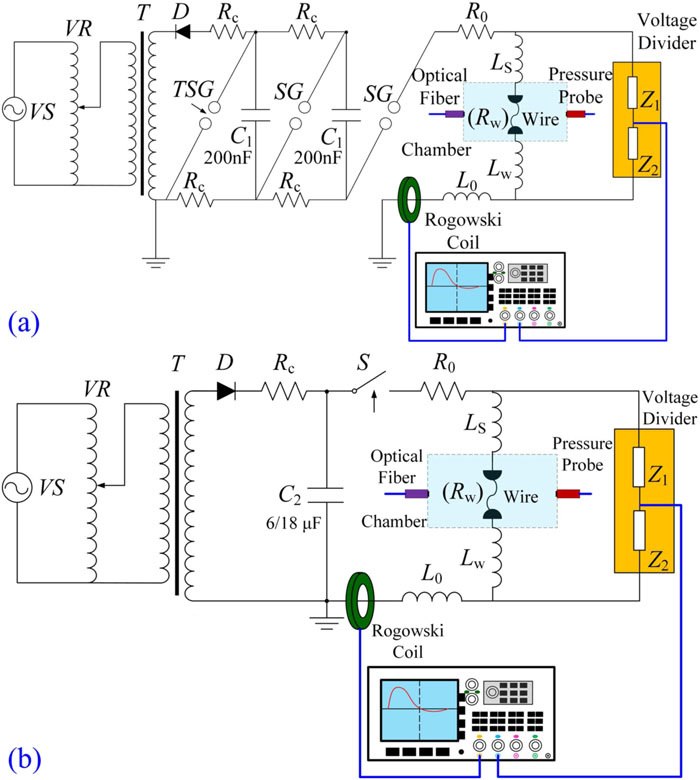
Fig. 1. Schematics of structure of SWE-2 when connected to source (a) 1 and (b) 2 or 3. Reproduced with permission from Han et al. , Rev. Sci. Instrum. 88 , 103504 (2017). Copyright 2017 AIP Publishing LLC.
As shown in
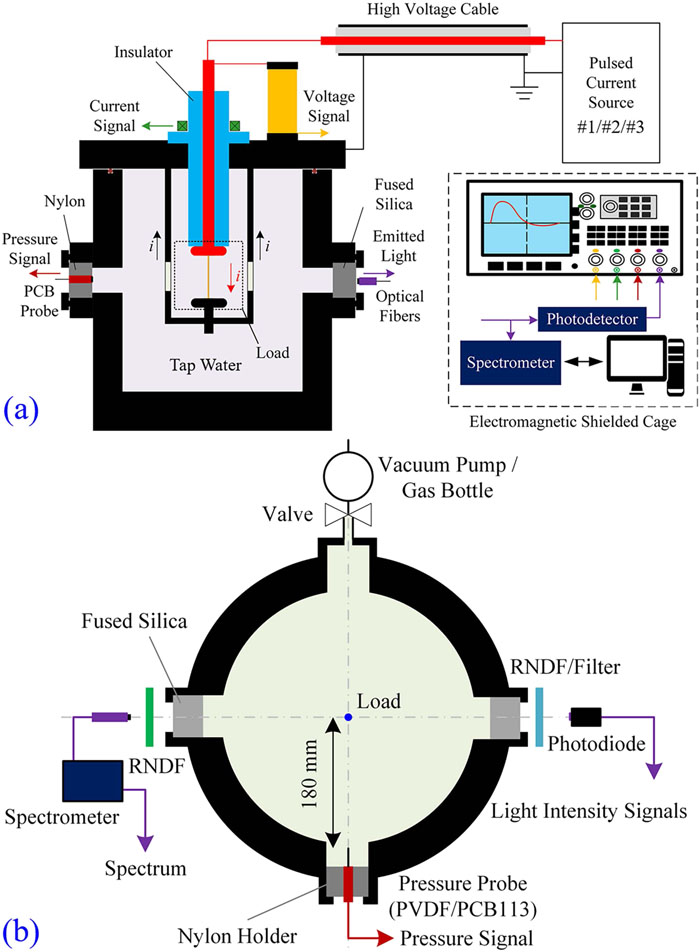
Fig. 2. Schematics of SWE-2: (a) connection of pulsed power source and chamber; (b) arrangement of diagnostic and auxiliary systems. Reproduced with permission from Han et al. , Rev. Sci. Instrum. 88 , 103504 (2017). Copyright 2017 AIP Publishing LLC.
2.2 B. Methods for extracting related electrical, optical, and mechanical parameters
Some of the detected data must be processed before being analyzed. The voltage signal U obtained via the probe is
For the optical emission spectroscopy, the nonlinear absorption effects are corrected as
For the SWs, the sharp front is difficult to detect, thereby causing distortion. Consequently, the pressure waveforms of the SWs must be reconstructed.
3 III. UEWE BEHAVIORS UNDER DIFFERENT DISCHARGE TYPE (MODE)
3.1 A. Concept of discharge type
Different combinations of wire and pulsed-current parameters result in different discharge types, namely distinct differences in phase transitions, optical emission, and SWs. In the 1950s, Chace and Moore
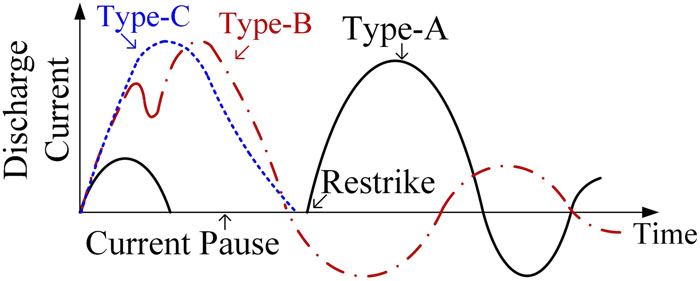
Fig. 3. Three representative discharge types of underwater electrical-wire explosion (UEWE) as represented by the current waveform.
The past two decades have seen great progress regarding the dynamics of exploding products, which helps understand the formation mechanisms of different discharge types. For example, Tkachenko et al.
3.2 B. Characteristics of UEWE under three typical discharge types
Although the physics of discharge type is understood relatively clearly in the laboratory, most investigations concern particular phenomena for particular purposes. For instance, the type-A current pause separates vaporization and breakdown (ionization) and so is common in some physical research. Type B often appears when metal plasma is focused. Because the majority of the stored energy goes into vaporizing the wire, type C is used is phase-transition studies and to generate strong SWs, which is important for SW physics and the properties of warm dense matter.
![Discharge parameters and light-intensity waveforms for UEWEs with four sizes of wire (labeled in the figures) under a stored energy of 500 J. Each row [(a) and (b), (c) and (d), (e) and (f), (g) and (h)] depicts the same discharge, but display different timescales of the x-coordinate. Reproduced with permission from Han et al., J. Appl. Phys. 122, 033302 (2017). Copyright 2017 AIP Publishing LLC.](/richHtml/mre/2020/5/4/047201/img_4.jpg)
Fig. 4. Discharge parameters and light-intensity waveforms for UEWEs with four sizes of wire (labeled in the figures) under a stored energy of 500 J. Each row [(a) and (b), (c) and (d), (e) and (f), (g) and (h)] depicts the same discharge, but display different timescales of the x-coordinate. Reproduced with permission from Han et al. , J. Appl. Phys. 122 , 033302 (2017). Copyright 2017 AIP Publishing LLC.
The peak and duration of the optical-emission waveforms are illustrated and summarized in
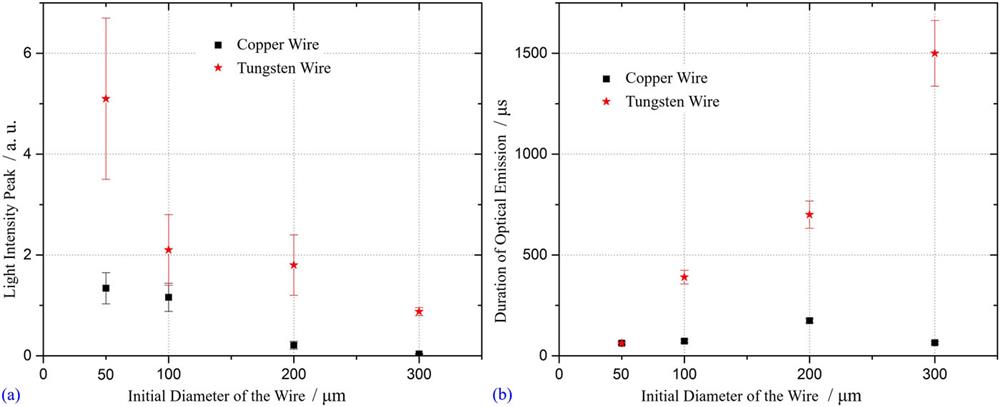
Fig. 5. (a) Peak and (b) duration for optical-emission process of Cu and W wire explosions with four sizes under a stored energy of 500 J. Reproduced with permission from Han et al. , J. Appl. Phys. 122 , 033302 (2017). Copyright 2017 AIP Publishing LLC.
For a deeper understanding of the above phenomenon, related time-integrated spectra of the above UEWEs are shown in

Fig. 6. Time-integrated spectra for Cu wire explosions. Reproduced with permission from Han et al. , J. Appl. Phys. 122 , 033302 (2017). Copyright 2017 AIP Publishing LLC.
The temporal evolution of the emission spectra is understood using narrow bandpass filters and photodiodes whose wavelengths are 402 nm, 480 nm, 523 nm, 530 nm, and 637 nm. The light intensities for those five wavelengths are shown in
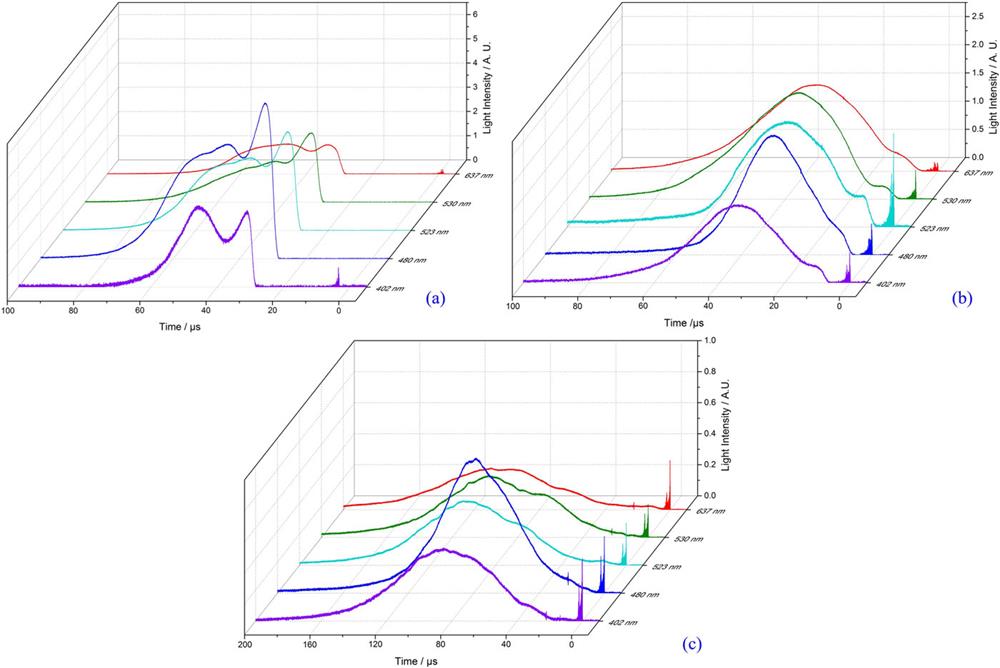
Fig. 7. Light intensity at five wavelengths in Cu wire explosion with d = (a) 50 µ m, (b) 100 µ m, and (c) 200 µ m. Reproduced with permission from Han et al. , J. Appl. Phys. 122 , 033302 (2017). Copyright 2017 AIP Publishing LLC.
In terms of the resulting SWs, the three discharge types lead to distinctly different features. The pressure waveforms associated with the discharge waveforms are shown in
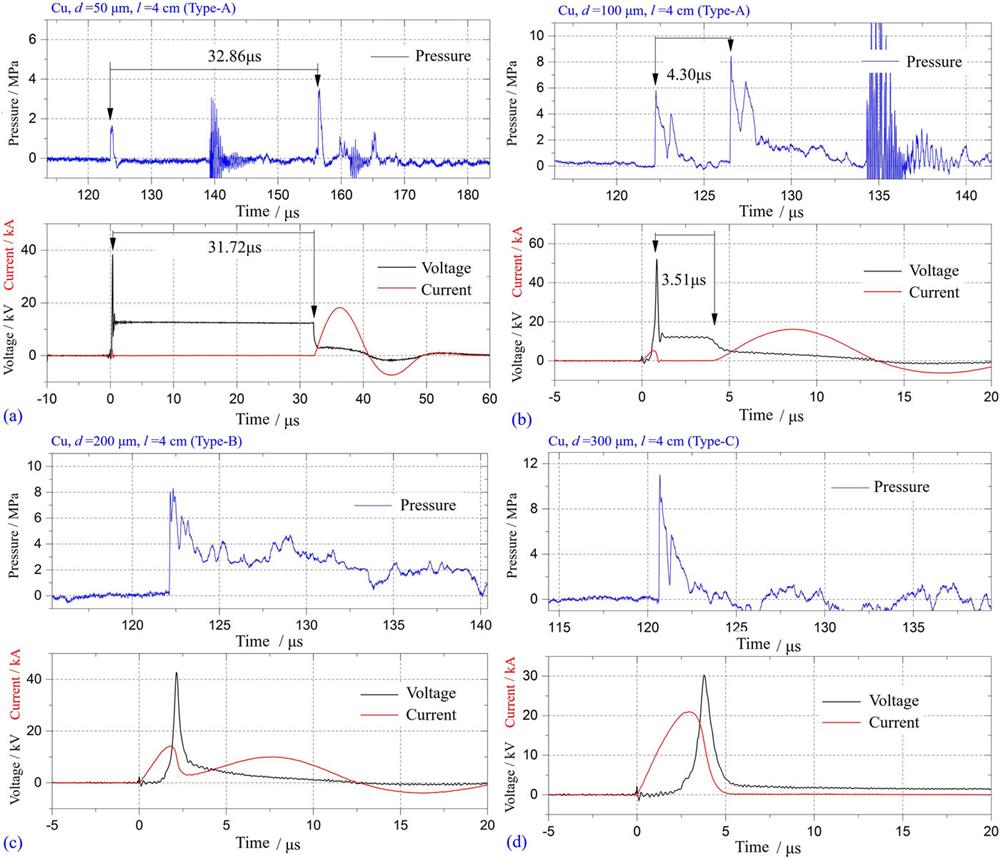
Fig. 8. Pressure waveforms of types (a) and (b) A, (c) B, and (d) C. Reproduced with permission from Han et al. , J. Appl. Phys. 122 , 033302 (2017). Copyright 2017 AIP Publishing LLC.
Combing the results of discharge, optical emission, and SW parameters gives a rough picture of the UEWE discharge type, which is determined mainly by how the DC ionization develops. The breakdown process is accompanied by optical emission and further DC expansion. From type A to C, a larger amount of energy is used in the vaporization stage, thereby resulting in stronger SWs and weaker optical emission.
Different discharge types have different purposes. Type-A discharges tend to be used only to investigate the effects of two successive SWs in the laboratory. For practical UEWE engineering, type B is suitable for igniting EMs, and type C generates the strongest SW for a given stored energy. Although most of the stored energy goes into vaporizing the wire under type C, the stored energy should exceed the atomization enthalpy for the particular wire material to prevent pseudo-explosion
4 IV. GENERATION PROCESS AND CHARACTERISTICS OF SHOCK WAVES
4.1 A. Brief review of shock-wave generation in UEWE
It is well known that the SW in a UEWE is produced by the fast expansion of the DC. In 2005, Grinenko et al.
In general, how a UEWE generates SWs is clear in the laboratory, but the relationships between the electrical and SW parameters are yet to be established systematically. For practical engineering, estimating the SW parameters roughly based on the input parameters (stored energy, wire configuration, etc.) would be of great importance. In our previous work, we have sought to connect the input parameters and the output SW parameters, and the main results are as follows.
4.2 B. Experimental verification of generation mechanism
It is known that SWs are generated by the continuous expansion of the DC during a wire explosion. However, the contribution of the vaporization or ionization process to the formation of SWs is not clear. Therefore, an experiment was designed to verify the importance of vaporization for SWs.
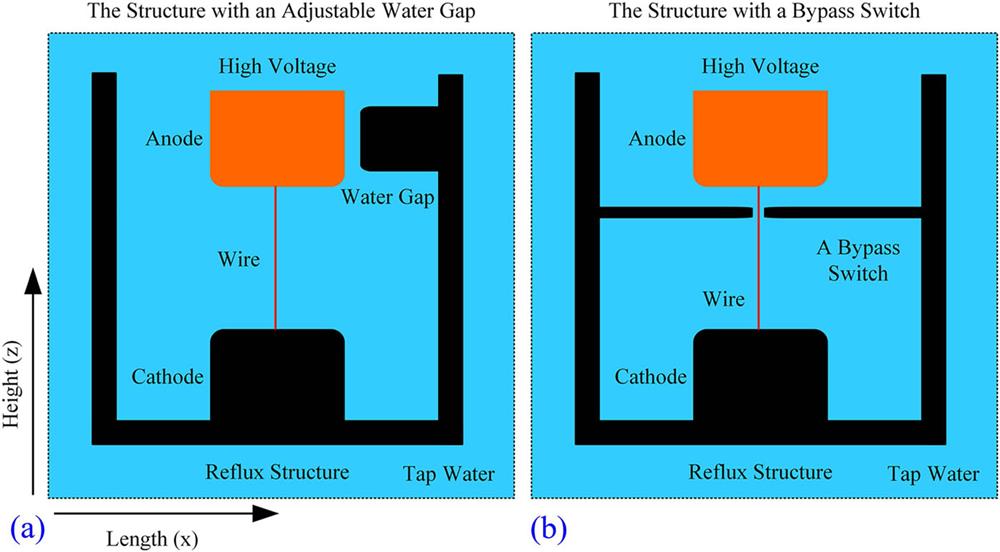
Fig. 9. Two types of energy-bypass device. Reproduced with permission from Han et al. , Phys. Plasmas 24 , 063511 (2017). Copyright 2017 AIP Publishing LLC.
To facilitate comparison with previous experiments, a metal Cu wire with a diameter of 200 µm and a length of 4 cm was selected, and the experiment was performed under a stored energy of 500 J. This metal wire was chosen because the type-B discharge involves all stages (from solid to plasma) of an electrical explosion. Given that the load voltage of a Cu-wire electrical explosion in its early stage increases monotonically with time, adjusting the gap distance of the water gap is a convenient way to achieve breakdown at different moments before the voltage peak. However, the high dielectric strength of water makes it difficult to achieve a breakdown in the plasma stage at low voltage. Therefore, using a bypass switch in conjunction with the water gap allows the wire load to be bypassed at different stages of the entire discharge process. The corresponding voltage and current waveforms are shown in
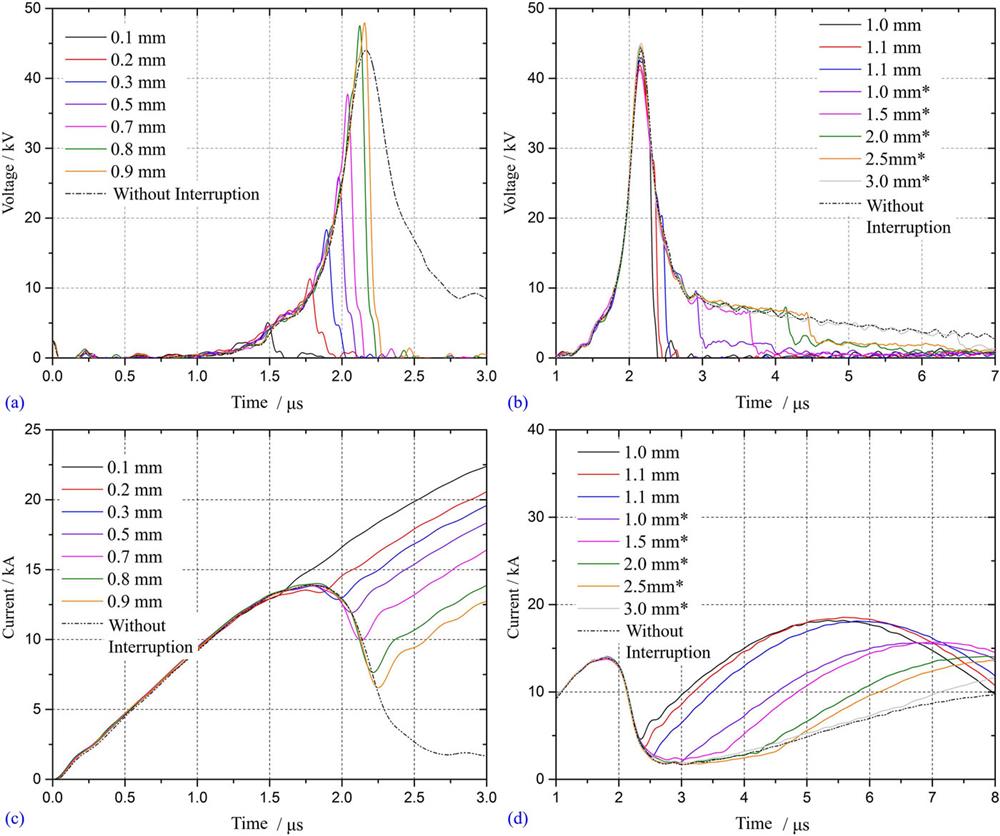
Fig. 10. Typical (a) and (b) voltage and (c) and (d) current waveforms when water gap or bypass switch acts at different moments. Reproduced with permission from Han et al. , Phys. Plasmas 24 , 063511 (2017). Copyright 2017 AIP Publishing LLC.
The light-intensity waveforms from the above experiment are shown in
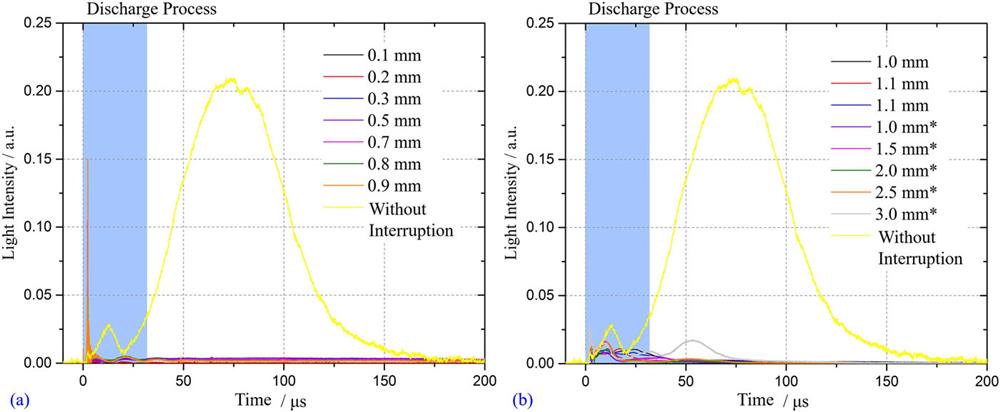
Fig. 11. Typical light-intensity waveforms when water gap or bypass switch acts at different moments. Reproduced with permission from Han et al. , Phys. Plasmas 24 , 063511 (2017). Copyright 2017 AIP Publishing LLC.
The pressure waveforms of the SWs in the above experiment are shown in
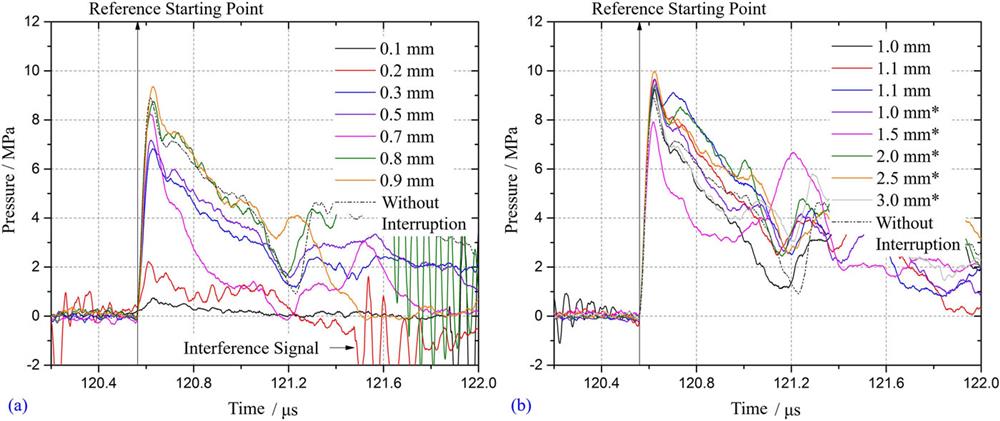
Fig. 12. Typical pressure waveforms of shock waves (SWs) when water gap or bypass switch acts at different moments. Reproduced with permission from Han et al. , Phys. Plasmas 24 , 063511 (2017). Copyright 2017 AIP Publishing LLC.
To conclude, the explosion occurring after vaporization is significant for the SW strength. First, the energy deposition for phase transitions matters for SW only when the explosion happens. Once the explosion occurs, the DC expands rapidly and relatively strong SWs are generated. Second, the energy deposition from the moment of explosion to the voltage collapse helps to maintain the DC volume expansion, thereby increasing the SW energy until the SW front separates from the DC. Third, the energy deposited into the developed plasma channel may have a limited effect on the SWs, but it is significant for the plasma radiation. The results above verify the contribution that vaporization makes to the generation of strong SWs in a UEWE. For a given stored energy, the strongest SWs arise when the electrical energy is absorbed before the voltage collapse (type C).
4.3 C. Characteristics of underwater shock waves
The aforementioned experiments revealed how light radiation and SWs are generated in a UEWE. Nevertheless, predicting the SW parameters is a sophisticated process, even with the help of numerical simulations. Given that SWs are extremely important in engineering, further characterization research is needed. To facilitate comparison with previous experiments, a 4-cm-long metal Cu wire with a diameter of 300 µm was selected, and the stored energy (charging voltage) in the system for electrical explosion was 500 J (−12.9 kV), 675 J (−15.0 kV), 1016 J (−18.4 kV), 1348 J (−21.2 kV), 1688 J (−23.7 kV), 2028 J (−26.0 kV), 2363 J (−28.1 kV), and 2700 J (−30.0 kV).
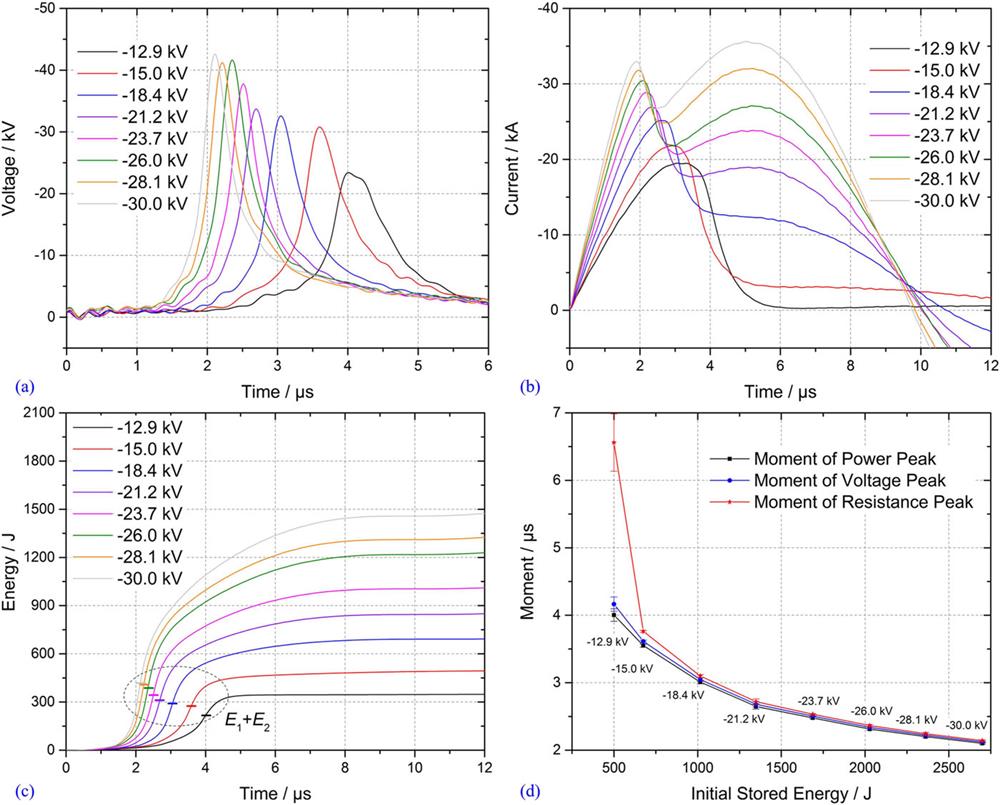
Fig. 13. Typical (a) voltage, (b) current, (c) deposition energy, and (d) timings of power, voltage, and resistance peaks for exploding a 300-μ m-diameter, 4-cm-long Cu wire under a stored energy of from 500 J (−12.9 kV) to 2700 J (−30.0 kV). The marks in (c) show the deposited energy at the voltage peak. Reproduced with permission from Han et al. , Phys. Plasmas 24 , 093506 (2017). Copyright 2017 AIP Publishing LLC.
The complexity of the electrical explosion process means that as well as the voltage and current waveforms, one must also analyze the relationships between the discharge and SW parameters, for example the influence of energy deposition at different stages of the UEWE. The energy deposition at different stages is defined as

Fig. 14. (a) Deposited energy and (b) energy deposition efficiency vs initial stored energy. Reproduced with permission from Han et al. , Phys. Plasmas 24 , 093506 (2017). Copyright 2017 AIP Publishing LLC.
We also introduce the parameter Π from the research by Grinenko et al.,
Except for the almost linear relationship between the impulse Jimpulse and the parameter Π in

Fig. 15. Relationships between SW parameters and electrical parameters of UEWE. Reproduced with permission from Han et al. , Phys. Plasmas 24 , 093506 (2017). Copyright 2017 AIP Publishing LLC.
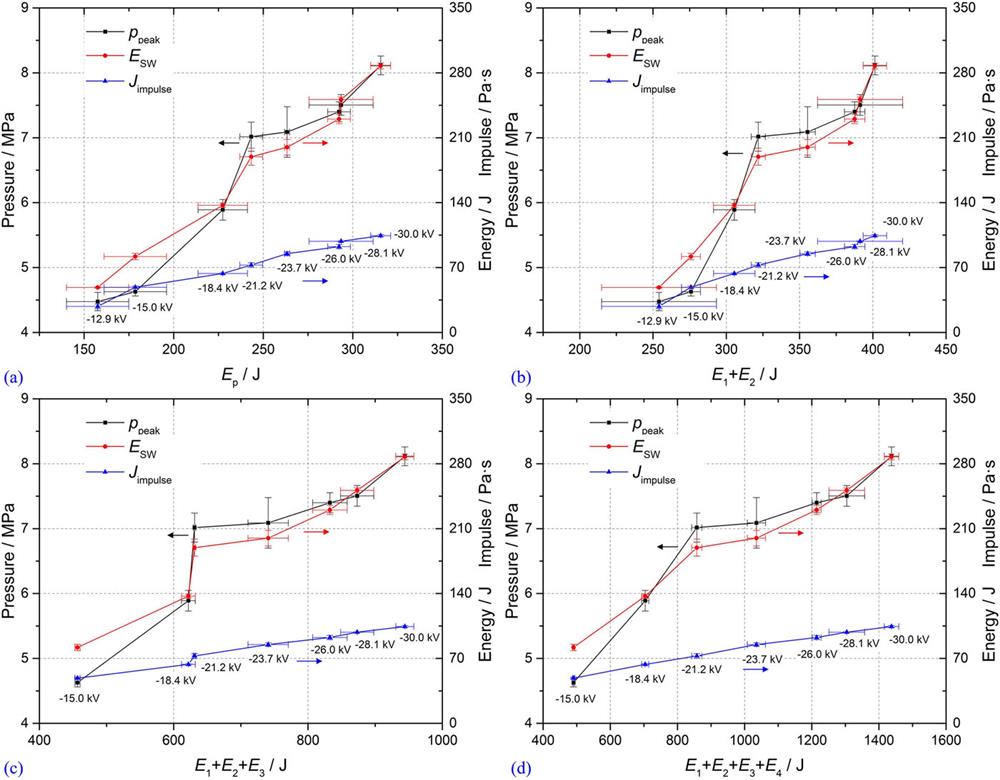
Fig. 16. Relationship between SW parameters and deposition energy in different stages of eight types of UEWE. Reproduced with permission from Han et al. , Phys. Plasmas 24 , 093506 (2017). Copyright 2017 AIP Publishing LLC.
The above results suggest that there is no simple relationship between the SW and electrical parameters. Although the impulse Jimpulse appears to have a linear relationship with Π or the energy deposition E1 + E2 + E3 + E4, the pressure distribution of the SW does not follow a simple pattern. Accordingly, the DC expansion undergoes a complex variation with increasing stored energy. The SW amplitude should be determined mainly by the deposition energy when the SW front separates from the DC. The deposition energy from the moment of separation to the zero-crossing point of the current goes mainly into generating optical radiation and slowing the attenuation of the expansion rate of the explosion product. Increasing the stored energy from 500 J to 2700 J results in nonlinear enhancement of energy deposition in different stages non-uniformly. Therefore, the DC expansion and SWs cannot be described simply by any of the discharge parameters.
4.4 D. Empirical approach for estimating shock-wave parameters (Cu wire)
Although there is no simple relationship between the SW and electrical parameters, the SW strength tends to increase with increasing stored energy. Therefore, empirical formulas could be used to describe the relationships between SW and discharge parameters, and thus one could estimate the SWs from the measured discharge parameters, which would be significant for practical engineering.

Fig. 17. Typical waveforms and stage division of discharge process. Reproduced with permission from Yao et al. , Phys. Plasmas 26 , 093502 (2017). Copyright 2017 AIP Publishing LLC.
5 V. INFLUENCE OF WIRE MATERIAL AND AMBIENT MEDIUM
Understanding UEWE with other metal materials would be useful for practical engineering, and UEWE in wells or oceans faces a water medium of high conductivity, pressure, and/or temperature. Therefore, in this section we present some experimental studies on the influences of the wire material and the ambient medium.
5.2 A. Exploding different metal wires in water
In Z-pinch research, the wire material plays an extremely important role in the subsequent X-ray radiation. Romanova et al.
1. UEWE of group 1
In this group, 4-cm-long Al, Cu, Ag, and Au wires with a diameter of 200 µm were exploded under a stored energy of 500 J. The discharge parameters and optical-emission intensity are illustrated in

Fig. 18. Voltage, current, and light-intensity waveforms for Al, Cu, Ag, and Au wire explosions with a wire diameter of 200 µ m (a) and (b), with different timescale of x-axis. Reproduced with permission from Han et al. , J. Appl. Phys. 124 , 043302 (2018). Copyright 2018 AIP Publishing LLC.
The measured time-integrated optical emission spectra of Al, Cu, Ag, and Au wire explosions are shown in
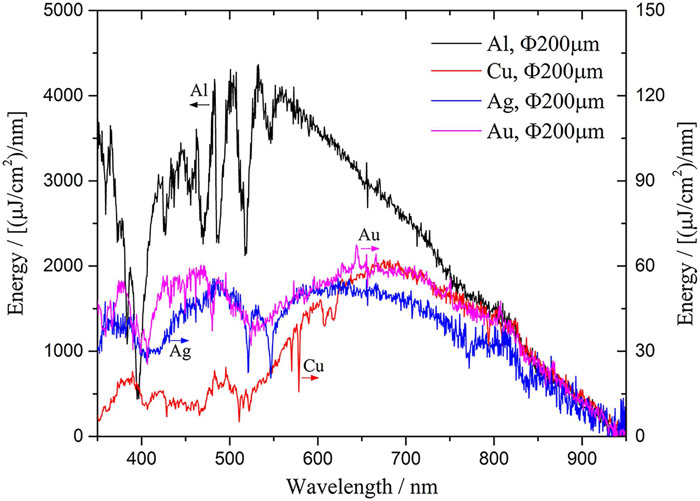
Fig. 19. Time-integrated spectra of Al, Cu, Ag, and Au explosions with a wire diameter of 200 µ m. Reproduced with permission from Han et al. , J. Appl. Phys. 124 , 043302 (2018). Copyright 2018 AIP Publishing LLC.

Fig. 20. Pressure waveforms of SWs for Al, Cu, Ag, and Au explosions with a wire diameter of 200 µ m. Reproduced with permission from Han et al. , J. Appl. Phys. 124 , 043302 (2018). Copyright 2018 AIP Publishing LLC.
2. UEWE of group 2
In this group, 4-cm-long Mo, Ta, and W wires with a diameter of 200 µm were exploded under a stored energy of 500 J. The discharge parameters and optical-emission intensity are shown in
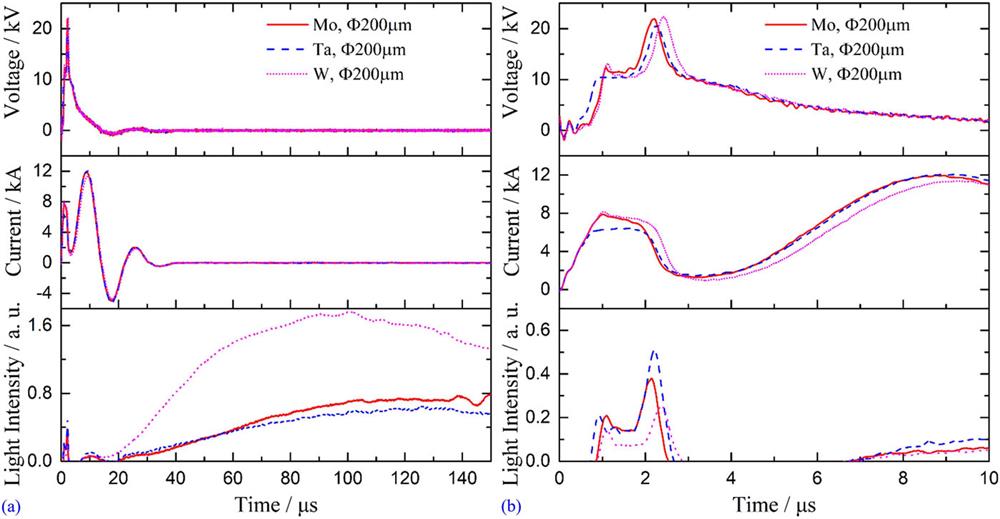
Fig. 21. Voltage, current, and light-intensity waveforms for Mo, Ta, and W explosions with a wire diameter of 200 µ m (a) and (b), with different timescale of x-axis. Reproduced with permission from Han et al. , J. Appl. Phys. 124 , 043302 (2018). Copyright 2018 AIP Publishing LLC.

Fig. 22. Time-integrated spectra of Mo, Ta, and W explosions with a wire diameter of 200 µ m. Reproduced with permission from Han et al. , J. Appl. Phys. 124 , 043302 (2018). Copyright 2018 AIP Publishing LLC.
3. UEWE of group 3
In this experiment, 4-cm-long Ti, Fe, and Pt wires with a diameter of 200 µm were evaluated under a stored energy of 500 J. The discharge parameters and optical-emission intensity are shown in
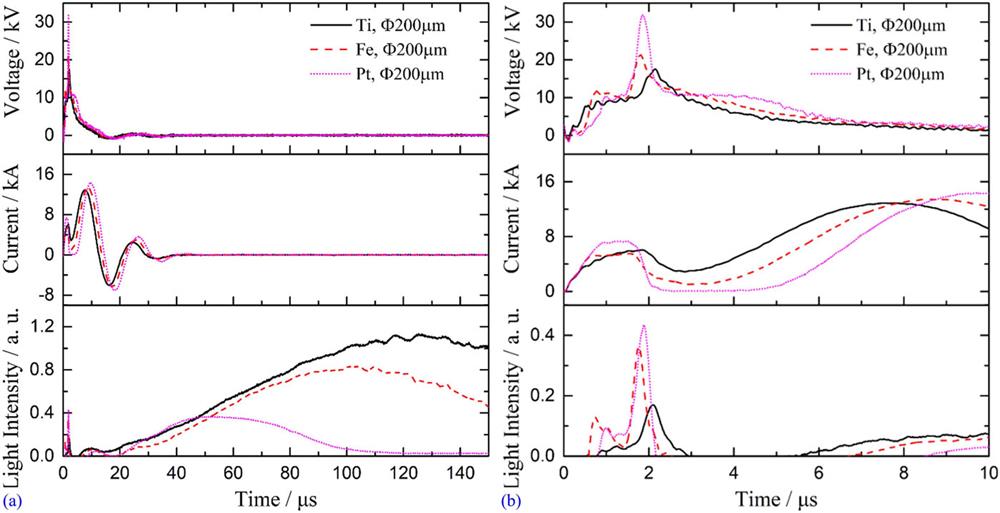
Fig. 23. Voltage, current, and light-intensity waveforms for Mo, Ta, and W explosions with a wire diameter of 200 µ m (a) and (b), with different timescale of x-axis. Reproduced with permission from Han et al. , J. Appl. Phys. 124 , 043302 (2018). Copyright 2018 AIP Publishing LLC.
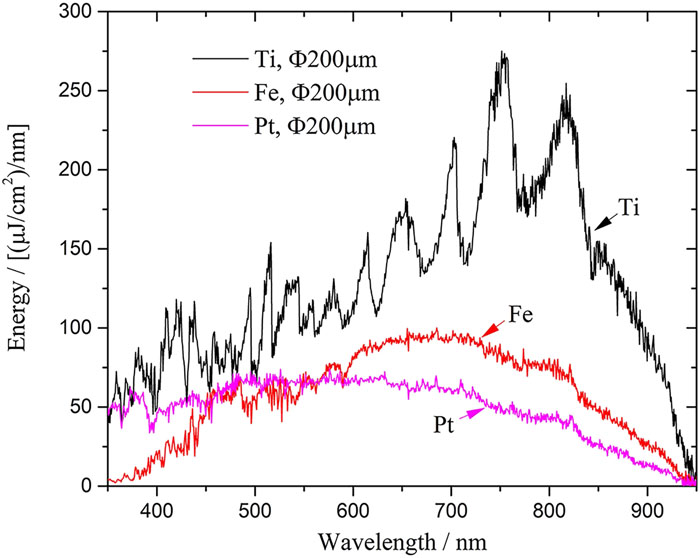
Fig. 24. Time-integrated spectra of Ti, Fe, and Pt explosions with a wire diameter of 200 µ m. Reproduced with permission from Han et al. , J. Appl. Phys. 124 , 043302 (2018). Copyright 2018 AIP Publishing LLC.
4. A brief summary
To aid and support the discussion, the experimental parameters for the shots are summarized in
Table 1. Summary of discharge parameters for metals in groups 1–3.
|
When comparing different metal wires of the same size, the effects of the material properties are obvious. Regarding the discharge properties, the group-1 metals have the highest voltage peak while the group-2 ones have the lowest. The differing electrical behavior is due to the electrical and thermophysical properties of the test materials. Accordingly, the energy-deposition characteristics show different patterns.

Fig. 25. Ratio (E 1 + E 2)/E atom for experiments with different wire materials. Reproduced with permission from Han et al. , J. Appl. Phys. 124 , 043302 (2018). Copyright 2018 AIP Publishing LLC.
5.3 B. Wire explosions in water in different states
In practical engineering, the liquid in the well often has higher conductivity, hydrostatic pressure, and temperature than those of the water used in laboratory tests. Therefore, how the water parameters influence the behavior of a UEWE must be investigated. An experiment was designed to study a Cu wire explosion (200-µm diameter, 4-cm length) in water with different conductivity, hydrostatic pressure, and temperature.
1. Exploding a wire in salt solutions (184 µS/cm–7410 µS/cm)
To study the influence of water conductivity, NaCl was used to prepare different salt solutions.
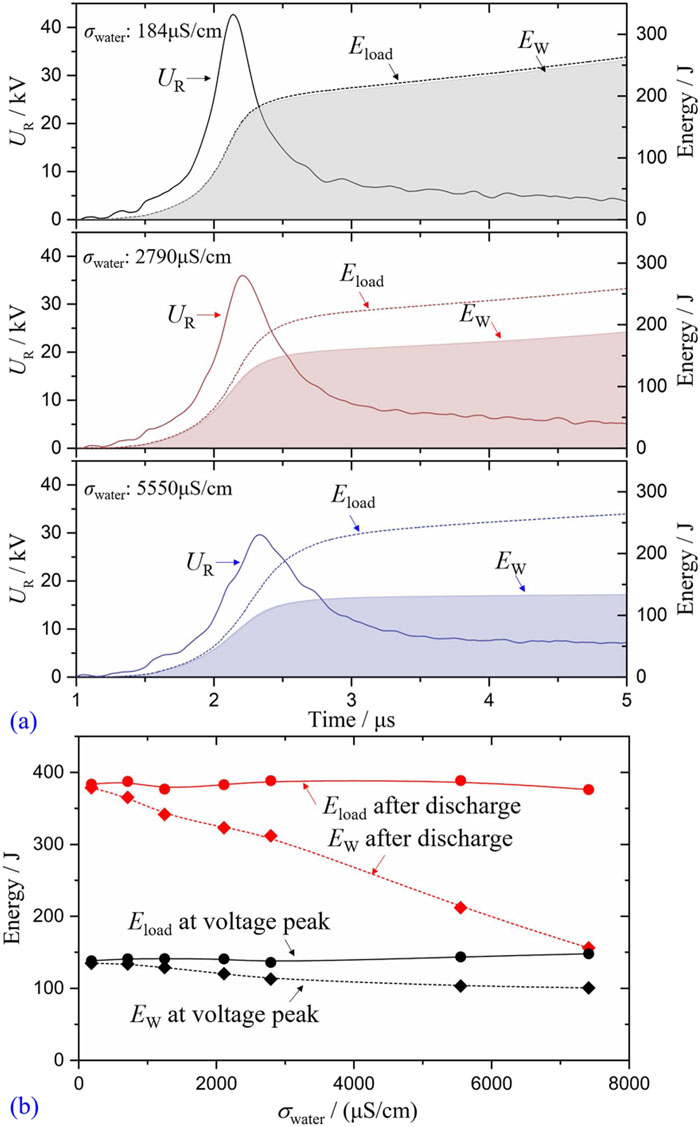
Fig. 26. Energy (a) deposition process and (b) variation tendency for explosion of a 200-μ m-diameter, 4-cm-long Cu wire in different salt solutions. Reproduced with permission from Han et al. , Eur. Phys. J. Plus 135 , 50 (2020). Copyright 2020 Springer Nature.
2. Exploding a wire under different hydrostatic pressures (0.1 MPa–0.9 MPa)
In tap water (184 µS/cm, 283 K), wires of 4-cm-long and 200-μm-diameter were exploded under hydrostatic (absolute) pressures of 0.1 MPa, 0.5 MPa, and 0.9 MPa.
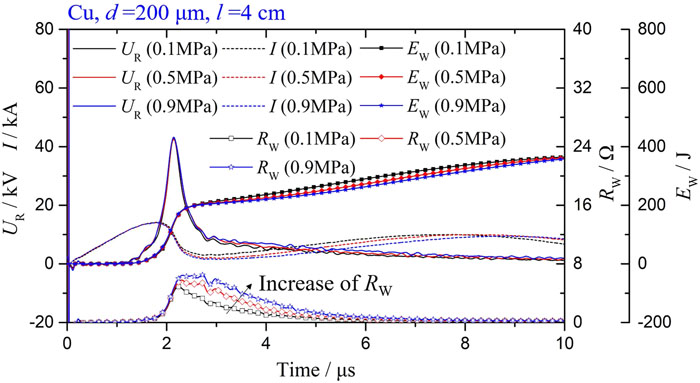
Fig. 27. Load voltage U R, circuit current I , deposited energy E W, and load resistance R W waveforms (representative) for exploding a 200-μ m-diameter, 4-cm-long Cu wire under different hydrostatic pressures. Reproduced with permission from Han et al. , Eur. Phys. J. Plus 135 , 50 (2020). Copyright 2020 Springer Nature.
3. Exploding a wire under different temperatures (283 K–323 K)
In tap water (184 µS/cm, 0.1 MPa), wires of 4-cm-long and 200-μm-diameter were exploded at temperatures of 283 K, 303 K, and 323 K.
6 VI. INTENSIFICATION AND REGULATION OF SHOCK WAVES
Pre-setting a wire between the two electrodes of the water gap (UEWE) can provide an initial channel for the discharge current, help reduce pre-breakdown energy loss, improve the discharge stability, and improve the SW energy conversion efficiency.
Facing the demand for stronger SWs to crack reservoirs in practical engineering,

Fig. 28. (a) Structure and real object of an energetic material (EM) load. (b) Schematic of experimental setup with an EM load. Reproduced with permission from Han et al. , J. Appl. Phys. 125 , 153302 (2019). Copyright 2019 AIP Publishing LLC.
With different fillers, a group of EM loads is exploded in a 2-m-diameter chamber. The SW parameters are summarized in

Fig. 29. Variations of SW parameters (a) p peak and p peak/Δt rise, (b) t p and J impulse, and (c) E SW and η . EM-1 to EM-6 are filled with NH4NO3, NH4ClO4, and Al powders, and with different dimensions. Reproduced from with permission Han et al. , J. Appl. Phys. 125 , 153302 (2019). Copyright 2019 AIP Publishing LLC.
The experimental results in
As well as EM loads, wire-array explosions in water have become a research focus recently.
7 VII. FRACTURING EFFECT OF SHOCK WAVES VIA WIRE EXPLOSION
As mentioned in Sec.
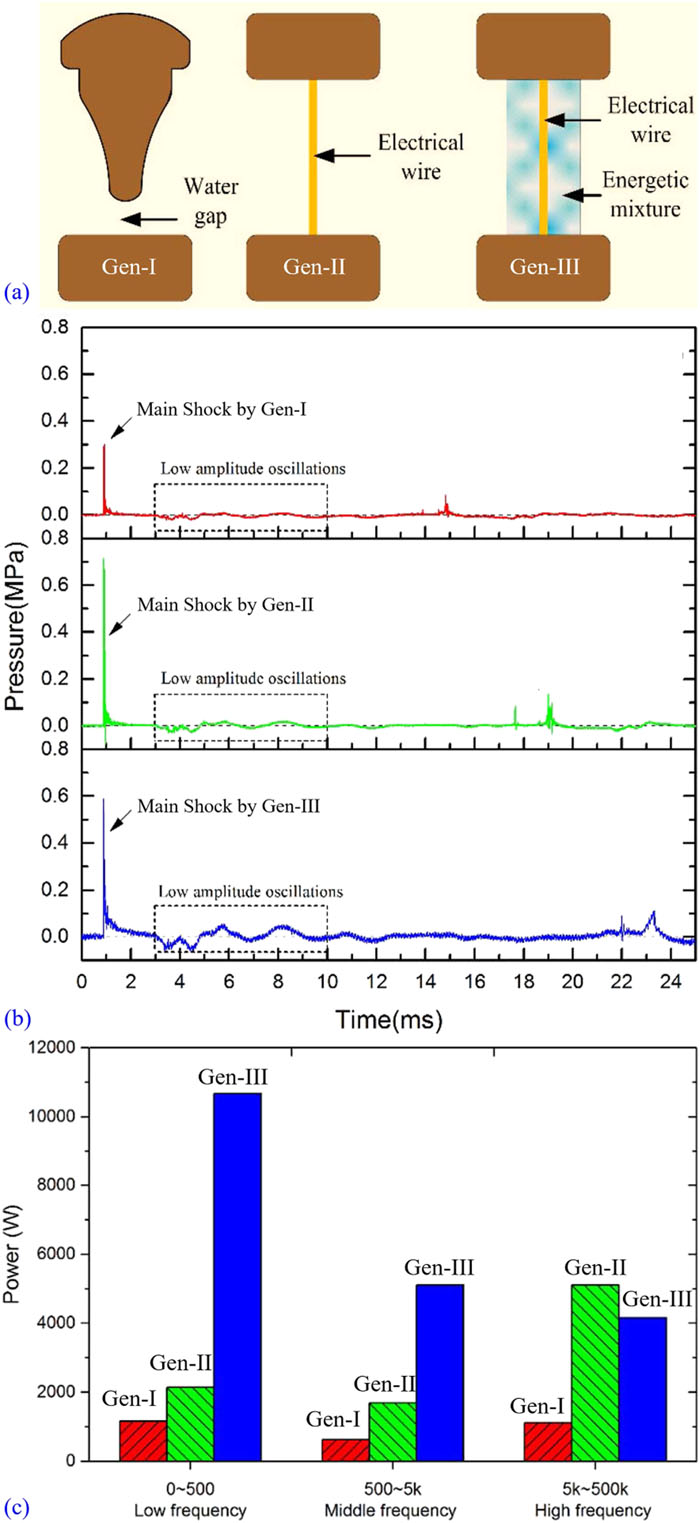
Fig. 30. (a) Schematics of three generations of underwater SW source proposed by our group. (b) Underwater SWs generated by a water gap (Gen-I), an exploding wire (Gen-II), and an EM load (Gen-III). (c) Power distribution within different frequency domains. Reproduced with permission from Zhou et al. , IEEE Trans. Plasma Sci. 43 , 4017–4023 (2015). Copyright 2015 IEEE.
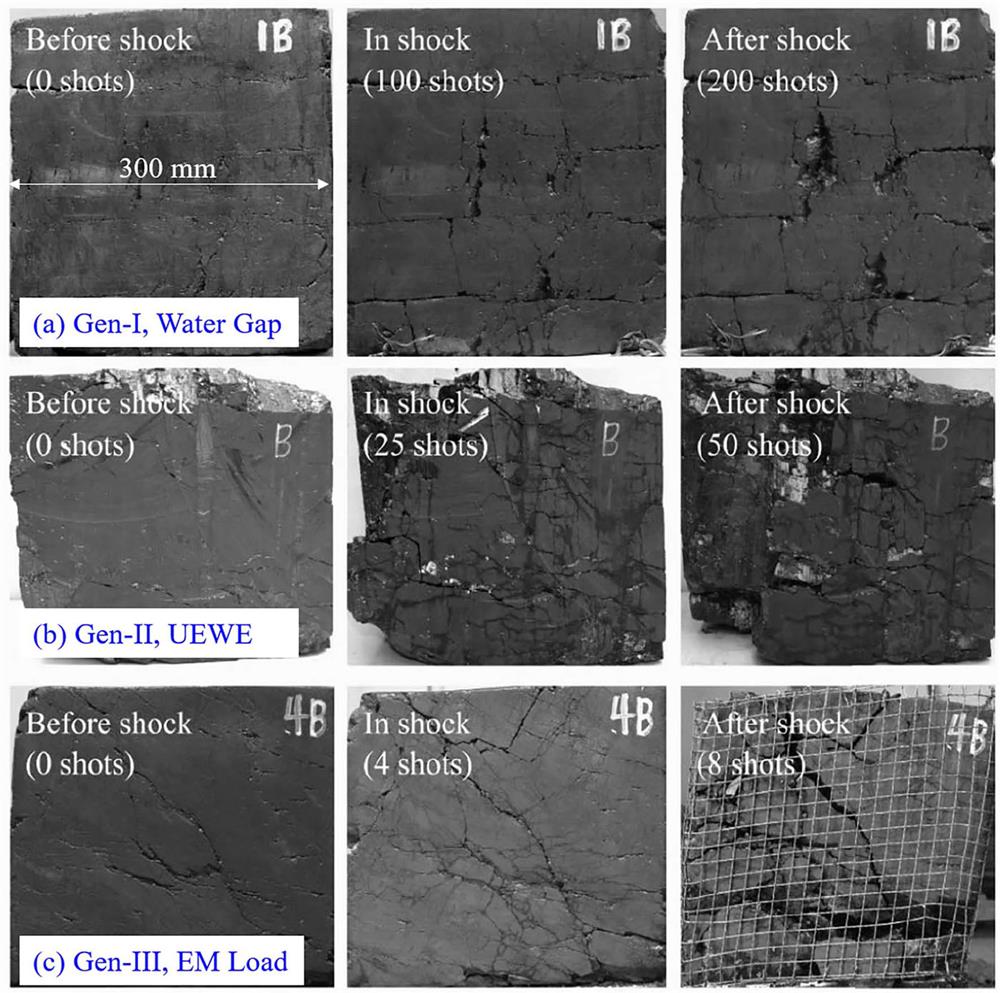
Fig. 31. Fracturing effects of three types of SW source on coal cube specimens. Reproduced with permission from Zhou et al. , IEEE Trans. Plasma Sci. 43 , 4017–4023 (2015). Copyright 2015 IEEE.
The results for the fracturing effect show that the EM load is the most effective of the three approaches. Note that UEWE generates dense and uniform cracks because of its abundance of high-frequency SWs. Therefore, in the past few years, we have made many efforts to apply UEWE and EM loads in practical engineering, including developing equipment and engineering practices, among other aspects. To date, China has seen much progress in exploiting coalbed methane and shale gas, among other sources, based on UEWE or EM loads.
To conclude, various experimental studies of UEWE have been conducted, and this review has summarized and presented a large amount of data on SW characteristics. Those data have supported dozens of engineering practices, which would also be helpful for the applications generating underwater SWs with similar methods, such as sound sources, mechanical processing, etc. However, the phenomenon of UEWE is still far from fully understood. Actually, we encountered many interesting phenomena and unsolved problems when dealing with the experiments. The main characteristics, difficulties, and challenges associated with this technology are listed in
Table 2. Main conclusions from previous studies and existing problems of UEWE for reservoir-stimulation technology.
|
8 VIII. CONCLUSIONS
A review of our recent experimental work concerning UEWE aimed at reservoir-stimulation technology was presented. Facing the complex working conditions in practical applications, the characteristics of optical emission and SWs were investigated systematically, which supports the development of reservoir-stimulation technology based on UEWE. The main findings are as follows.
First, the features of UEWE under different discharge types were clarified. Different combinations of metal wire and pulsed power source lead to different discharge types and phenomena. Three typical discharge modes are realized by the electrical explosion of 4-cm-long Cu and W wires with diameters of 50 µm–300 µm under a stored energy of 500 J: type A features a current pause, type B features a periodically oscillating circuit current, and type B features an aperiodic circuit current. Experimental results revealed that the fine metal wire (the wire with smaller diameter) easily meets type A, where no ionization occurs after the explosion and the secondary breakdown eventually occurs with the expansion of the explosion product, resulting in strong optical radiation and two successive SWs. With increasing diameter of the metal wire, the current pause gradually disappears. If the remaining energy after the explosion is sufficient, UEWE will follow type B, accompanied by strong light radiation and strong SWs. If the remaining energy is insufficient, then UEWE will follow type C, resulting in weak light radiation and strong SWs.
Second, the generation mechanisms and characteristics of SWs were researched. Two energy-bypass devices were designed to stop the energy injection into the wire at different stages of UEWE. For optical radiation, if the load is short-circuited before the plasma process, then the measured optical radiation is very weak. By contrast, if the load is bypassed after the formation of plasmas, then strong optical radiation is measured. For the SWs, if the load is short-circuited before the explosion, then the measured SW amplitude is below 2.2 MPa, otherwise the SW amplitude is above 6.8 MPa. The above experiment proves that the main source of optical emission is plasma. The SW generation mechanism is the expansion of the DC. This expansion is affected by the energy-deposition process. For loads of the same specification, as the system’s energy storage increases, the SW parameters are positively related to the electrical parameters such as deposition energy. The strength of the SW is determined mainly by the energy deposited before the SW front separates from the DC.
Third, UEWEs with different metallic materials were compared and analyzed. For Nb, Mo, Ta, W, and Re, a large amount of deposited energy is used for phase transitions, and the energy deposited before the voltage peak is 1–1.5 times the atomization enthalpy. For Cu, Ag, and Au, the deposited energy is usually more than twice the atomization enthalpy. Those differences make non-refractory metal wires generate SWs that are notably stronger those generated by refractory ones. Chemically active metals such as Al, Ti, and Fe react with water during the electrical-explosion process, producing intense light radiation. The heat released by the reactions can accelerate the phase transitions. Metals with high ionization energy, such as Au and Pt, are relatively difficult to ionize, thus the light radiation and SWs are weak.
Fourth, to clarify how the surrounding medium affects a wire explosion, this research compared and analyzed wire explosions in air and water. Water is effective at impeding the expansion and ionization of explosion products, resulting in large deposited energy and weak optical emission from high-density plasmas. As for the water parameters, with increasing water conductivity, a higher proportion of the circuit current passed through the salt solution, resulting in a lower energy-deposition rate and phase-transition process. As for hydrostatic pressure, it was found that the ambient pressure had a slight effect on the fast stage of DC expansion. However, the applied hydrostatic pressure could suppress the later stage of DC expansion, resulting in a higher DC resistance. Conversely, temperatures of 283 K–323 K have a limited effect on the wire-explosion process.
Finally, as a promising method for strengthening or regulating SWs, EMs ignited by wire explosion were investigated. The energy of the SWs increased from less than 200 J (UEWE) to more than 8 kJ (high explosive) under the same stored energy. Specifically, the type, quality, and geometric size of the EM have significant effects on the SWs. The strongest SWs were produced by high-explosive loads. Ordinary EMs such as ammonium nitrate mainly lengthen the tp and impulse of SWs, when compared with the bare-wire case. The larger the energetic load mass, the larger the duration and impulse of the SWs. The larger the diameter of the EM load, the slower the SW head.
Article Outline
Ruoyu Han, Jiawei Wu, Haibin Zhou, Yongmin Zhang, Aici Qiu, Jiaqi Yan, Weidong Ding, Chen Li, Chenyang Zhang, Jiting Ouyang. Experiments on the characteristics of underwater electrical wire explosions for reservoir stimulation[J]. Matter and Radiation at Extremes, 2020, 5(4): 047201.