Smooth Archimedean-spiral ring waveguide for cold atomic gyroscope
Download: 959次
There has been widespread interest in developing an atomic gyroscope, as the theoretical sensitivity of an atomic gyroscope corresponds to an improvement by a factor of
The challenges to realizing a ring waveguide on an atom chip for a guided atomic gyroscope include avoiding the trapping perturbation[32,33] caused by the input and output ports of the rings and creating a non-zero field waveguide at the minimum. When the ultracold atoms propagate through the waveguide and are close to the zero magnetic field points, the atoms confined in the waveguide may be lost by Majorana spin flips into untrapped magnetic sub-states[34], which limit the sensitivity of the atomic gyroscope due to their short lifetime and low coherence time. To create a non-zero field ring waveguide at the minimum, one way is to use an azimuthal magnetic bias field[32], which can be generated simply by a straight current-carrying wire perpendicular to the chip surface and that passes through the center of the ring waveguide. The challenge is the limited size of the ring waveguide on the chip of just a few millimeters. The other way is to apply a time-averaged orbiting potential (TOP), which is generated by a rapidly oscillating trap field[35]. The TOP technique[34] was used originally to lift the quadrupole trap minimum from zero, which has led to the first observation of a Bose–Einstein condensate (BEC)[36]. By using the TOP technique, a BEC has been produced in a ring waveguide generated by coils[24], in which the time-averaged potential has been generated by varying the currents of the coils.
In this Letter, we propose to investigate a ring waveguide on a single-layer atom chip for a cold atomic gyroscope. The wire configuration is based on an Archimedean spiral[37]. The wire layout avoids the trapping perturbation caused by the input and output ports, resulting in an enclosed guiding loop for neutral atoms in the weak field-seeking states. However, there are two symmetrical zero magnetic field points in the ring waveguide minimum, which is an inherent characteristic of the Archimedean spiral ring magnetic potential. We use a rotation bias field to lift the ring waveguide minimum from zero and smooth the waveguide at the same time. The location of the waveguide is immune to the magnetic variations, as it is only determined by the wire layout of the atom chip, resulting in a very stable and smooth ring waveguide.
The wire layout to generate the ring potential on the chip is shown in Fig.
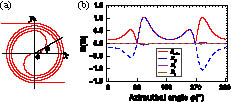
Fig. 1. (a) The wire layout to generate a ring potential on the chip and the definition of the azimuthal angle . The radius of the ring potential is 1000 μm and the separation distance between the successive turnings is 110 μm, which determines the height location of the ring potential. (b) The minimum of the magnetic field at a fixed azimuthal angle with a dc current of 1 A. (red line) is the magnetic field strength of the minimum. (purple line), (blue dashed line), and (brown dashed line) are the three components of in the cylindrical coordinate system. The variation of the minimum of the magnetic field is about 1 G.
In order to eliminate Majorana spin-flip losses coursed by zero magnetic field points and smooth the minimum of the ring waveguide, we employ a rotation bias field to create a time-orbiting potential trap. In the
To produce a time-averaged potential for the atoms, the rotation frequency
For an appropriate value of the rotation frequency
After applying the rotation bias magnetic field, the minimum of the ring waveguide is non-zero and the ring waveguide is smoothed, as shown in Fig.

Fig. 2. Time-averaged magnetic field of the minimum at a fixed azimuthal angle with a rotation bias magnetic field. The magnitude of the bias magnetic field is 2 G. The variation of the minimum of magnetic field is 0.14 G.
Figure

Fig. 3. Locus of the position of the instantaneous zero points (blue lines), known as the circle of death, and the time-averaged minimum points (red line). The circles of death are two circles around the minimum of the ring waveguide. The image in the center shows a zoomed-in view of a slice of the circles of death. The radius of death is about 10 μm.
To further illuminate the time-averaged potential, we investigate the value of magnitude
Figure

Fig. 4. Variation of the characteristics of the time-averaged potential as a function of the magnitude of the bias field . (a) The filed gradient in the direction (blue line) and the radius of death (red dashed line) are represented by and , respectively. (b) The trap of depth (blue line) and the variation of the minimum of magnetic field (red dashed line) are represented by and , respectively.
We will now briefly discuss the experimental scheme for the atomic gyroscope on the atom chip. After evaporative cooling, the cold atoms or BEC are loaded into the ring waveguide[29], and a sequence of two standing-wave square pulses are applied to split the cold atoms into two symmetrical parts with a well-defined initial momentum[41]. Subsequently, the two symmetrical parts start to freely propagate in the ring waveguide in opposite directions. When the atoms return to the loading point, a second double-square-pulse beam splitter is applied to recombine the two parts. Then we can detect the number of atoms that carry a phase shift to measure the rotation.
In conclusion, we propose a scheme to create a ring waveguide based on Archimedean-spiral wires on an atom chip for an atomic gyroscope. The Archimedean-spiral wires can create an enclosed ring waveguide which avoids the trapping perturbation caused by the input and output ports. With a rotation bias field, a time-averaged potential is created to lift the minimum of the ring waveguide from zero and smooth the minimum. The location of the ring waveguide on the atom chip is only determined by the wire layout. The optimal value of 3 G of the bias magnetic field is obtained, which keeps the waveguide smooth and sufficiently deep for ultracold atoms. The Archimedean-spiral ring waveguide offers the prospect of developing a compact and portable atomic gyroscope.
[1]
[2]
[3]
[4]
[5]
[6]
[7]
[8]
[9]
[10]
[11]
[12]
[13]
[14]
[15]
[16]
[17]
[18]
[19]
[20]
[21]
[22]
[23]
[24]
[25]
[26]
[27]
[28]
[29]
[30]
[31]
[32]
[33]
[34]
[35]
[36]
[37]
[38]
[39]
[40]
[41]
Xiaojun Jiang, Xiaolin Li, Haichao Zhang, Yuzhu Wang. Smooth Archimedean-spiral ring waveguide for cold atomic gyroscope[J]. Chinese Optics Letters, 2016, 14(7): 070201.