基于数值仿真的Al2O3陶瓷激光选区熔化表面微观组织形成机理研究
下载: 947次
1 引言
陶瓷材料具有熔点和硬度高以及耐磨性、抗氧化性、化学稳定性好等优点[1],但其加工比较困难,磨削、车削、研磨、注浆、蚀刻等传统加工技术难以加工复杂的高精度陶瓷零部件,这严重制约了陶瓷材料在工业生产中的应用。激光选区熔化(SLM)技术能够直接成形高精度的复杂零件,是高效、低成本加工陶瓷零部件的潜在制造技术。目前,陶瓷SLM技术刚刚起步[2-3],其成形机理及成形过程中的特殊现象亟待解释。
本课题组在前期的研究中发现,在Al2O3陶瓷材料SLM成形试件表面存在条状、胞状等凝固结构[4],这种凝固结构与常见的Al2O3晶粒不同,虽然它们大小相近,但是这种凝固组织呈长条状,分布有序,方向一致,长度相似。本课题组研究认为这是熔池热毛细对流造成的一种对流快速凝固现象,并分析了凝固界面流动形式与激光功率、扫描速度、预热温度的关系。熔池对流是影响SLM成形过程的重要因素之一,由于流动对传热、传质的影响,熔池对流驱动气泡在熔池内运动,影响熔池形貌[5],并进一步影响试件的表面质量[6-7]。
Takaichi等[8]、Cherry等[9]和Kruth等[10]在Co-Cr-Mo、316L材料的SLM试件表面上也发现了类似的微米级胞状组织结构,金属凝固组织通常比陶瓷凝固组织更细小,且形态更多变。除长条状,六角胞状组织也较为常见。Prashanth等[11]和周鑫[12]认为该组织与传统工艺例如铸造形成的亚晶粒在尺度、空间分布上不同,且具有流动特性,并与贝纳德-马兰戈尼对流有关。此外,Prashanth等还利用贝纳德-马兰戈尼失稳理论和颗粒聚集-结构形成原理解释了金属复合材料亚稳态六角形细胞状结构现象,提出了复合材料产生这种细胞结构的条件。周鑫分析了现有合金实验中出现的胞状、伸长胞状和条状组织的形成原因,认为微熔池非平衡快速凝固与非线性热毛细对流存在相互耦合作用。
本文在上述研究的基础上,采用数值仿真方法,研究了Al2O3陶瓷实验中出现的条纹凝固组织的形成机理。
2 模型的建立及参数设置
2.1 数值仿真模型
建立长方体模型,其尺寸为1.6 mm×1.6 mm×0.4 mm,设置六面体网格,网格大小为0.02 mm ×0.02 mm×0.02 mm,共有128000个单元。
SLM成形过程中的熔化凝固现象颇为复杂,整个瞬态过程涉及热源、粉末、气氛环境之间的热传导和热对流,以及凝固过程中的结晶潜热、熔池中因温差造成的热毛细对流等现象。为了在不影响准确性的前提下减小计算量,对模型做出以下基本假设:1)汽化带来的能量变化相比热传导和热对流都很小,所以忽略汽化;2)材料参数中的热导率、比热容、黏度随温度变化,其他物理参数如表面张力梯度、密度为常数;3)假设熔池自由表面固定,忽略材料熔化前为浆料,不区分基板和粉体。
2.2 控制方程及相变潜热的计算
根据流体力学理论设置控制方程:能量方程、动量方程、质量方程[13]。相变潜热Δ
式中:
流体分数定义在液相温度与固相温度之间从0到1均匀增长,即
式中:
2.3 初始化及边界条件
考虑到Al2O3陶瓷材料的激光束吸收长度数量级较大,因此使用体热源,其热生成率
式中:
初始温度应为环境温度
在热源加载面上,除了热源输入外,还有Al2O3陶瓷粉末与气氛的热辐射、热传导。表达式为
式中:
模型侧面的速度为零,故而其速度边界条件表达式为
式中:
模型侧面仅有陶瓷粉末与气氛的热传导,故而其温度边界条件表达式为
温差导致表面张力差,表面张力差导致马兰戈尼对流。马兰戈尼对流通过计算液体表面的切应力
式中:d
2.4 材料成分及物理参数
实验采用的Al2O3粉末成分如
表 1. Al2O3的化学成分
Table 1. Chemical compositions of Al2O3
|
仿真时采用纯Al2O3的物理参数,比热容
表 2. Al2O3的部分参数
Table 2. Part parameters of Al2O3
|
当温度大于熔点时,Al2O3变成液体状,采用非接触式载体技术测量的结果来计算Al2O3的表面张力
式中:
2.5 仿真及实验工艺参数
采用南京理工大学自主研发的陶瓷材料SLM实验平台进行实验。该设备配备了IPG公司生产的单模连续型光纤激光器以及感应加热装置,如
仿真和实验采用相同的工艺参数,激光扫描方式为单向多道,具体参数值如
表 3. 仿真及实验工艺参数
Table 3. Simulation and experiment processing parameters
|
3 结果分析与凝固组织形成机理讨论
3.1 温度场与流场的仿真结果

图 3. 仿真结果。(a)总体温度场;(b)流场剖面图
Fig. 3. Simulation results. (a) Overall temperature field; (b) cross-sectional profile of flow field
![仿真和实验中的熔池尺寸。 (a)激光功率为140 W,扫描速度为70 mm·s-1时,熔池的形状和温度云图;(b)激光功率为140 W,扫描速度为70 mm·s-1时,试件的SEM图[4] ;(c)激光功率为140 W时,不同扫描速度下的熔池数值仿真尺寸;(d)扫描速度为90 mm·s-1时,不同激光功率下的熔池数值仿真尺寸](/richHtml/zgjg/2019/46/2/0202002/img_4.jpg)
图 4. 仿真和实验中的熔池尺寸。 (a)激光功率为140 W,扫描速度为70 mm·s-1时,熔池的形状和温度云图;(b)激光功率为140 W,扫描速度为70 mm·s-1时,试件的SEM图[4] ;(c)激光功率为140 W时,不同扫描速度下的熔池数值仿真尺寸;(d)扫描速度为90 mm·s-1时,不同激光功率下的熔池数值仿真尺寸
Fig. 4. Molten pool dimensions in simulation and experiment. (a) Geometry of molten pool and temperature cloud map for laser power of 140 W and scanning speed of 70 mm·s-1; (b) SEM image of specimen for laser power of 140 W and scanning speed of 70 mm·s-1; (c) molten pool sizes in numerical simulation for different scanning speeds but same laser power of 140 W; (d) molten pool sizes in numerical simulation for different laser powers but same scanning speed of 90 mm·s-1
根据以上仿真得到的温度场,对温度求导获得任意一点的各方向的温度梯度值。在与
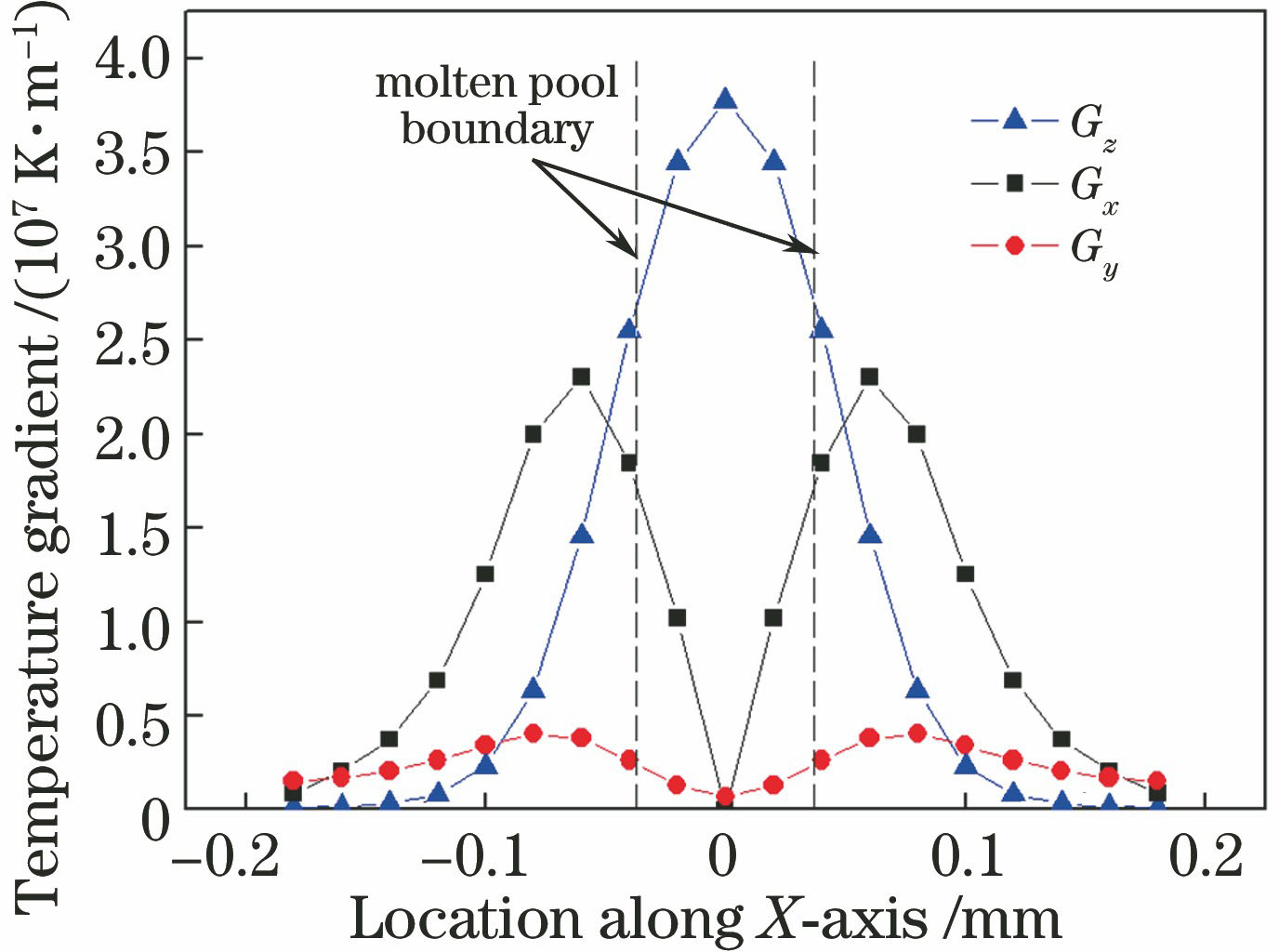
图 5. 当P=140 W, v=70 mm·s-1时, z=0、y=0.5474 mm路径上的温度梯度
Fig. 5. Temperature gradient along path at z=0 and y=0.5474 mm for P=140 W and v=70 mm·s-1
3.2 实验结果

图 6. 不同工艺参数下试件的扫描电镜图
Fig. 6. SEM images of parts fabricated at different process parameters
![陶瓷试件表面的凝固组织[4]。(a) 条状组织;(b)指状组织](/richHtml/zgjg/2019/46/2/0202002/img_7.jpg)
图 7. 陶瓷试件表面的凝固组织[4]。(a) 条状组织;(b)指状组织
Fig. 7. Surface solidification structure on the ceramic specimen. (a) Stripy structure; (b) finger-shaped structure
3.3 Al2O3陶瓷熔池对流对凝固组织的影响
熔池内的对流十分复杂,存在马兰戈尼对流[20]和贝纳德-马兰戈尼对流[21]。马兰戈尼对流由温度梯度驱动,如
贝纳德-马兰戈尼对流形式与水平马兰戈尼数
式中:
针对陶瓷SLM成形实验中的熔池对流现象,由仿真获得400 K预热温度、不同工艺参数下Al2O3陶瓷材料SLM成形温度云图和温度梯度曲线,并计算了马兰戈尼数:液态Al2O3的热扩散率为1.06×10-6 m2·s-1,动力黏度为0.03 N·s·m-2。以熔池中心(温度最高的节点)为交点画十字,在该十字上共取15个点,用仿真结果计算各个工艺参数下的
采用同样的计算方法,可以得到激光功率为200 W,扫描速度为90 mm·s-1时,不同预热温度下的
表 4. 不同激光功率及扫描速度下的Mhor和Mver
Table 4. Mhor and Mver obtained at different laser powers and scanning speeds
|
表 5. 不同预热温度下的Mhor和Mver
Table 5. Mhor and Mver obtained at different preheating temperatures
|
根据Mizev[23]的观点,马兰戈尼数(
将
由

图 9. 不同激光功率及扫描速度下表面微观组织的预测结果。 (a)熔池表面对流预测结果;(b)对应的流动形式图
Fig. 9. Prediction results of surface microstructure at different laser powers and scanning speeds. (a) Prediction results of convection on molten pool surface; (b) corresponding flow pattern

图 10. 不同预热温度下表面微观组织的预测结果。(a)熔池表面对流的预测结果;(b)对应的流动形式图
Fig. 10. Prediction results of surface microstructure at different preheating temperatures. (a) Prediction results of convection on molten pool surface; (b) corresponding flow pattern
以上由对流快速凝固形成的组织会对表面平整度产生影响。由此,分析认为,对于Al2O3陶瓷SLM试件,高激光功率、慢扫描速度、高预热温度都可能会导致失稳的贝纳德-马兰戈尼对流,从而导致试件表面凹凸不平。因此,在选择这三个工艺参数时,不仅要考虑粉末的充分熔化,以及避免球化、裂纹等,还要考虑控制上述微观组织,低激光功率、快扫描速度、低预热温度有助于避免失稳态贝纳德-马兰戈尼对流。
4 结论
通过数值仿真计算获得了Al2O3陶瓷SLM成形的温度场和流场,并进一步计算了不同工艺参数条件下的马兰戈尼数,结合实验研究阐明了Al2O3陶瓷SLM成形表面凝固组织的形成机理,获得以下结论:
1) Al2O3陶瓷SLM成形具备发生贝纳德-马兰戈尼表面失稳状态的条件,当扫描速度为90 mm·s-1、激光功率为140~200 W时,或者扫描速度为60~90 mm·s-1、激光功率为140 W时,发生条状滚卷式对流。随着激光能量减小,马兰戈尼数逐渐靠近稳态区,条状滚卷式对流逐渐减弱。
2) 基板预热能够改变贝纳德-马兰戈尼对流状态,随着预热温度升高,条状滚卷式对流逐渐向流动的贝纳德花转变。
3) 低激光功率、快扫描速度、低预热温度有助于避免失稳态贝纳德-马兰戈尼对流。
[1] Surendranathan AO. An introduction to ceramics and refractories[M]. Boca Raton: CRC Press, 2014: 7- 18.
Surendranathan AO. An introduction to ceramics and refractories[M]. Boca Raton: CRC Press, 2014: 7- 18.
[2] Bourell D, Kruth J P, Leu M, et al. Materials for additive manufacturing[J]. CIRP Annals, 2017, 66(2): 659-681.
Bourell D, Kruth J P, Leu M, et al. Materials for additive manufacturing[J]. CIRP Annals, 2017, 66(2): 659-681.
[3] 张凯, 刘婷婷, 廖文和, 等. 氧化铝陶瓷激光选区熔化成形实验[J]. 中国激光, 2016, 43(10): 1002007.
张凯, 刘婷婷, 廖文和, 等. 氧化铝陶瓷激光选区熔化成形实验[J]. 中国激光, 2016, 43(10): 1002007.
[10] Kruth JP, BadrossamayM, YasaE, et al. Part and material properties in selective laser melting of metals[C]. International Symposium on Electromachining, 2010: 3- 14.
Kruth JP, BadrossamayM, YasaE, et al. Part and material properties in selective laser melting of metals[C]. International Symposium on Electromachining, 2010: 3- 14.
[12] 周鑫. 激光选区熔化微尺度熔池特性与凝固微观组织[D]. 北京: 清华大学, 2016: 79- 98.
周鑫. 激光选区熔化微尺度熔池特性与凝固微观组织[D]. 北京: 清华大学, 2016: 79- 98.
ZhouX. Research on micro-scale melt pool characteristics and solidified microstructures in selective laser melting[D]. Beijing: Tsinghua University, 2016: 79- 98
ZhouX. Research on micro-scale melt pool characteristics and solidified microstructures in selective laser melting[D]. Beijing: Tsinghua University, 2016: 79- 98
[15] 戴冬华, 顾冬冬, 李雅莉, 等. 选区激光熔化W-Cu复合体系熔池熔体运动行为的数值模拟[J]. 中国激光, 2013, 40(11): 1103001.
戴冬华, 顾冬冬, 李雅莉, 等. 选区激光熔化W-Cu复合体系熔池熔体运动行为的数值模拟[J]. 中国激光, 2013, 40(11): 1103001.
[16] 张凯, 刘婷婷, 廖文和, 等. 氧化铝激光选区熔化温度场模拟[J]. 硅酸盐学报, 2017, 45(12): 1825-1832.
张凯, 刘婷婷, 廖文和, 等. 氧化铝激光选区熔化温度场模拟[J]. 硅酸盐学报, 2017, 45(12): 1825-1832.
[18] 张佩璜, 唱润忠, 魏臻, 等. 550~2400 K温区α-氧化铝焓值、熔点和熔化热的实验测定[J]. 计量学报, 1986( 2): 33- 39.
张佩璜, 唱润忠, 魏臻, 等. 550~2400 K温区α-氧化铝焓值、熔点和熔化热的实验测定[J]. 计量学报, 1986( 2): 33- 39.
Zhang PH, Chang RZ, WeiZ, et al. Experimental measurement of enthalpy, melting point and melting heat for α-alumina in the range from 550-2400 K[J]. Acta Metrologica Sinica, 1986( 2): 33- 39.
Zhang PH, Chang RZ, WeiZ, et al. Experimental measurement of enthalpy, melting point and melting heat for α-alumina in the range from 550-2400 K[J]. Acta Metrologica Sinica, 1986( 2): 33- 39.
[20] Kou S, Limmaneevichitr C, Wei P S. Oscillatory Marangoni flow: a fundamental study by conduction-mode laser spot welding[J]. Welding Journal, 2011, 90(12): 229-240.
Kou S, Limmaneevichitr C, Wei P S. Oscillatory Marangoni flow: a fundamental study by conduction-mode laser spot welding[J]. Welding Journal, 2011, 90(12): 229-240.
[22] Ueno I, Kurosawa T, Kawamura H. Thermocapillary convection in thin liquid layer with temperature gradient inclined to free surface[J]. Jasma Journal of the Japan Society of Microgravity Application, 2002, 19: 18-23.
Ueno I, Kurosawa T, Kawamura H. Thermocapillary convection in thin liquid layer with temperature gradient inclined to free surface[J]. Jasma Journal of the Japan Society of Microgravity Application, 2002, 19: 18-23.
Article Outline
马瑞芩, 张凯, 韦辉亮, 刘婷婷, 廖文和. 基于数值仿真的Al2O3陶瓷激光选区熔化表面微观组织形成机理研究[J]. 中国激光, 2019, 46(2): 0202002. Ruiqin Ma, Kai Zhang, Huiliang Wei, Tingting Liu, Wenhe Liao. Formation Mechanism of Surface Microstructure in Selective Laser Melting of Alumina Ceramic Based on Numerical Simulation[J]. Chinese Journal of Lasers, 2019, 46(2): 0202002.