Widely tunable, heterogeneously integrated quantum-dot O-band lasers on silicon
Download: 745次
1. INTRODUCTION
The rise in datacenter traffic is creating a demand for low-cost, low-power consumption, highly reliable optical transceivers. Silicon photonics is the only technology that can meet such demands. In the last decade, silicon photonic integrated circuits (PICs) have shown tremendous growth and have transitioned from research labs to products [1]. Today, silicon PICs are fabricated in state-of-the-art 300 mm pilot lines on silicon-on-insulator (SOI) wafers and take advantage of the highly mature fabrication processes developed for electronic integrated circuits (EICs). A key differentiating point between PICs and EICs is that the feature size for PICs does not scale with advanced nodes (e.g., the single-mode width of the waveguide is always the same). However, access to advanced technology nodes reduces defect density and process-dependent variations in silicon PICs. Since the advanced technology nodes are already developed for EICs, separate development for PICs is not needed thus saving costs. As a result, several foundry services are available across the globe that provide silicon PICs in the O-band (centered around 1310 nm) and the C-band (centered around 1550 nm). Low-loss waveguides and passive devices such as wavelength filters (ring resonators, Mach–Zehnder interferometers) and wavelength (de)multiplexers (arrayed waveguide gratings) are available in the portfolio of these foundries. Active devices in silicon PICs have also been realized by fabricating p-n junctions to form modulators or by depositing epitaxial germanium film to form detectors [2].
The only drawback for silicon PICs is the lack of a light source on account of silicon being an indirect bandgap material. On the other hand, III–V materials allow bandgap engineering, and light emission by electrical injection can be achieved. Quantum dots (QDs) are particularly attractive light emitters due to their atom-like density of states. This allows engineering of gain bandwidth and the peak emission wavelength by changing dot growth conditions [3]. QDs have a low linewidth enhancement factor (), which allows reducing the laser linewidth. Additionally, QD-based lasers are more insensitive to both feedback and defect density in comparison with quantum well (QW) lasers [4]. Therefore, integration of QD lasers on silicon PICs promises to bring the entire high-performing transceiver circuit on a single chip.
Two approaches to integrate QD lasers on silicon PICs are compatible with the wafer-scale process: epitaxial growth and heterogeneous integration. There has been extensive research on epitaxial growth of QD material on silicon substrates [57" target="_self" style="display: inline;">–
On the other hand, heterogeneous integration provides an attractive way of placing III–V gain material on SOI wafers. In this approach, III–V gain materials are bonded to a pre-patterned SOI wafer and then processed after substrate removal to form laser structures. Thanks to the similar refractive indices between III–V and silicon, light is efficiently transferred from the gain provided by III–V layer to passive silicon waveguides via adiabatic taper structures defined lithographically. Heterogeneous integration is a mature technology with 300 mm volume production feasibility, and several industrial players, e.g., Intel, Juniper Networks, and HPE, are producing or developing commercial transceivers based on it [1,11]. Silicon-nitride-based waveguides present an alternate to SOI waveguides and have ultralow loss [12]. External cavity narrow linewidth lasers have been realized using the hybrid [13] (where two chips are butt-coupled to each other) and heterogeneous approaches [14]. The hybrid approach is not scalable to wafer-level processing, and the heterogeneous approach requires multilevel wafer bonding, which has not yet been developed commercially. Additionally, due to low index contrast, silicon-nitride waveguide circuits have a large footprint compared with SOI waveguides. Therefore, heterogeneously integrated QD lasers on SOI wafers are the most promising candidates for reduced cost and size and more simple transceivers.
Several reports of single-mode lasers integrated on silicon, using both epitaxial growth and heterogeneous integration, working in the O-band have been published, including distributed feedback (DFB) lasers and tunable lasers. While DFB lasers can only be tuned within a few nm range, tunable lasers with wide-tuning range allow maintaining a flexible grid and reduce power consumption in tuning other transceiver network elements. This is essential in datacenters where a large number of transceivers are deployed.
In this paper, we report the first demonstration of widely tunable QD lasers heterogeneously integrated on SOI wafers operating in the O-band. Table
Table 1. Comparison of O-Band Single Wavelength Lasers on Silicon
|
2. LASER DESIGNS AND FABRICATION
The single-mode lasing is achieved by using two ring resonators in a Vernier scheme, where the two silicon passive rings have a slightly different free spectral range (FSR). This leads to an increase in the total FSR of the two-ring system allowing for wide tuning range. This scheme is employed to realize tunable lasers in several wavelength bands [23,24]. The III–V/silicon gain region is located between two mirrors. The back mirror consists of two ring resonators with the same waveguide width (500 nm) and slightly different radii (40 μm and 41.2 μm). The targeted FSR of each ring resonator is 1.746 and 1.683 nm, which gives a total Vernier FSR of 46.14 nm. The gap between the bus waveguide and the ring resonator is fixed at 265 nm, which corresponds to a 9% coupling coefficient. The front mirror is formed by a Sagnac loop, which is designed for 40% reflection. The 40% reflectivity of the front facet is a conservative value chosen to ensure we obtain enough reflections to compensate for cavity losses. This value can be improved in future-generation devices to achieve a balance between threshold current density and output optical power. The output from the front mirror is fed into a multimode interferometer (MMI) designed with a 15:85 splitting ratio. The 15% output of the MMI is connected to an on-chip avalanche photo-diode (APD) [25], and the 85% output is connected to a grating coupler. The insertion loss of the grating coupler was measured to be 10.5 dB. A silicon waveguide phase section is also included between the front mirror and the gain region for aligning the laser cavity modes with the ring modes. Both rings and the phase section have metal resistive heaters on them for thermo-optic tuning.
To increase the SMSR, a third filter in the form of a tunable Mach–Zehnder interferometer (MZI) is added to the Vernier ring geometry. The path length difference of the MZI is chosen such that the MZI FSR is twice the average ring FSR, so as to suppress the neighboring Vernier modes. More details on the operation principle of this device are discussed in Ref. [26]. A schematic diagram of both devices is shown in Figs.
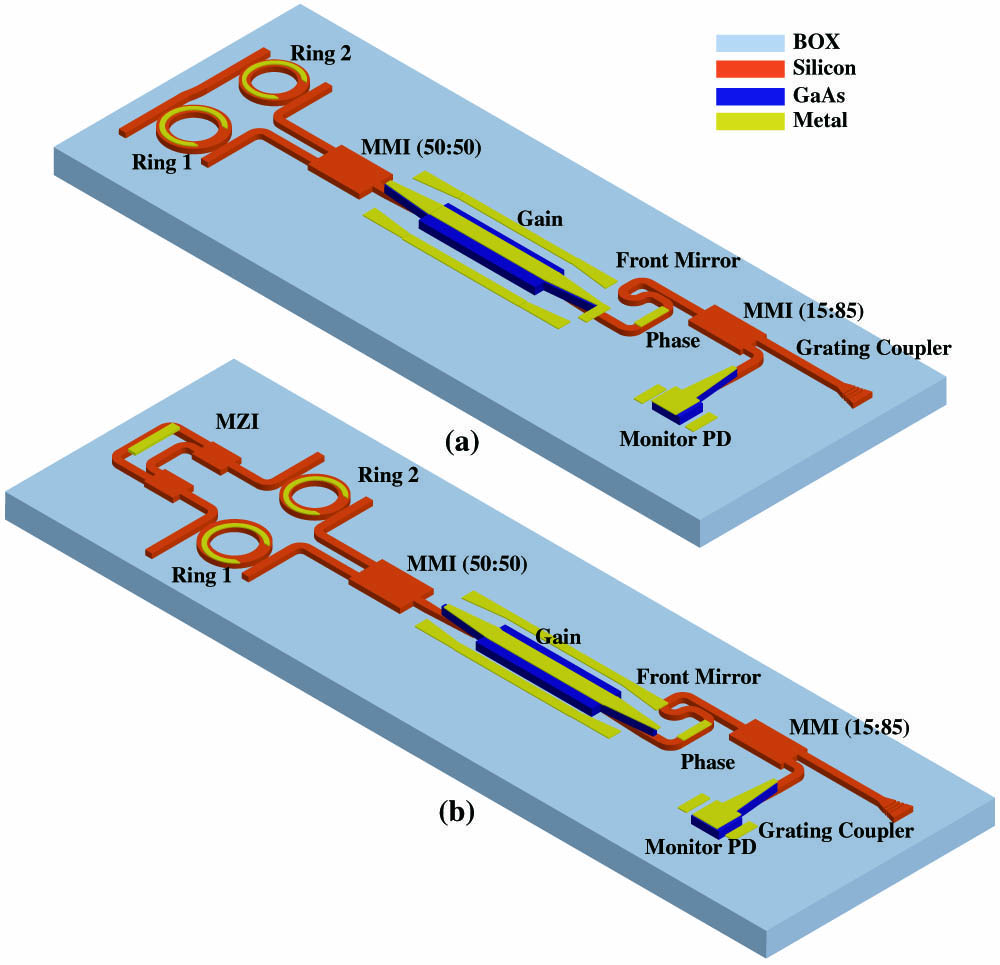
Fig. 1. Schematic diagram of (a) two-ring Vernier and (b) two-ring Vernier with MZI tunable laser. Electrical contacts are shown in gold.
The devices were fabricated on 100 mm wafers using the fabrication process described in Ref. [27]. The SOI wafer has a 400 nm thick silicon device layer and a 2 μm buried oxide layer. The gain material has a GaAs-based p-i-n structure with 8 QD layers. The gain region is 1.4 mm long, and the active region is 4 μm wide.
3. LASER CHARACTERIZATION
The devices were characterized on a temperature-controlled stage at 20°C. Electrical contacts were made using a probe card, and the output fiber was positioned using a closed-loop piezo electric stage. The output fiber was connected to an optical isolator followed by a 1:99 splitter. The 1% output was fed to a fiber alignment controller (Thorlabs K-Cube Nanotrak NIR), which monitors the piezo controls. The 99% output was connected to a 50:50 splitter feeding into a wave meter (Yokogawa AQ6150) and a power meter (Agilent 81635A). For subsequent tuning map measurements, the power meter was replaced by an optical spectrum analyzer (Yokogawa OSA AQ6370D). For linewidth measurements, the output going to the 50:50 splitter was instead connected to a polarization controller and polarization beam splitter (PBS), which was then connected to an OE waves linewidth measurement tool (OE4000). The other port of the PBS was fed to the OSA to monitor the SMSR.
3.2 A. Light–Current–Voltage Characteristics
The light–current–voltage (LIV) characteristics were obtained by sweeping the current in the gain region while monitoring the power collected in the fiber through the grating coupler. Figure

Fig. 2. (a) LIV characteristics of the Vernier ring laser when both rings are unbiased. (b) Spectral characteristics showing ring mode hops and cavity mode hops. (c) OSA trace at 290 mA drive current (curve has been truncated in the middle) showing both ground state and excited state lasing can be observed. (d) LIV characteristics of the Vernier ring laser when ring one is unbiased and ring two has 45.8 mW electrical power applied. (e) Spectral characteristics showing only cavity mode hops. (f) OSA trace at 290 mA drive current showing only ground-state lasing.
It is possible to operate the device in a pure ground state by optimally aligning the Vernier rings, allowing only a single reflection peak in the O-band. Figure
The LIV characteristics of the Vernier ring MZI laser are shown in Fig.

Fig. 3. (a) LIV characteristics of the Vernier ring MZI laser. (b) Spectral characteristics showing cavity mode hops.
The longitudinal mode spacing is used to calculate the total cavity length (3.812 mm for Vernier ring case and 4.741 mm for Vernier ring MZI case), which provides an estimate of the coupling coefficient (0.18 and 0.24, respectively). These higher coupling coefficients point toward a 50 nm smaller than designed gap between the waveguide and the rings.
3.3 B. Wavelength Tuning
The location of the lasing wavelength is determined by the reflection peak of the Vernier rings. Either of the rings can be tuned to choose the lasing wavelength. This effect is shown in Figs.

Fig. 4. Lasing wavelength as a function of the applied heater power for (a) the first ring and (b) the second ring.
To evaluate the laser performance completely, all of the tuning elements in the laser cavity need to be optimized. This was achieved by applying electrical power to the heaters on both rings sequentially in steps of 1 mW (while the gain current was kept at a constant value of 300 mA). For each point, the phase section was tuned from 0 to 60 mW in 1 mW steps while monitoring the APD current. These power values were necessary to achieve a full phase shift with some margin. The phase section was then set to maximize the APD photocurrent, and a spectral analysis was done using the wave meter to determine the number of lasing wavelengths quickly. A slower, high-resolution OSA scan was taken only for single-mode points. Obtaining the tuning map of the Vernier ring MZI laser is more complex than of the Vernier ring laser, as there are four tuning elements. The tuning algorithm was the same as that for the Vernier ring laser, except that both MZI and phase were sequentially tuned to maximize APD photocurrent. This tuning algorithm was executed using MATLAB scripts and Keithley SMU units. For more optimized tuning, a cycle of sequentially adjusting each heater should be iterated several times due to thermal cross-talk among the tuning elements. However, due to the large dimensional tuning space of the heaters (3D for two rings and phase and 4D with MZI) and also the limited speed of GPIB protocols, only one cycle was used for each data point. Tuning all the thermal phase tuners together can maintain a mode-hop-free operation in a short wavelength range dependent on the performance of the thermal phase shifter. The tuning map and SMSR are shown in Figs.

Fig. 6. Vernier ring laser: (a) automatically measured spectra over the entire tuning range and (b) manually optimized spectrum at a single point showing 52 dB SMSR.
For the Vernier ring MZI laser, the tuning range increases to 53 nm, and the overall SMSR also improves as compared with the Vernier ring laser, which is a direct result of the additional wavelength filtering provided by the MZI. The corresponding spectra after normalizing the grating coupler response is shown in Fig.
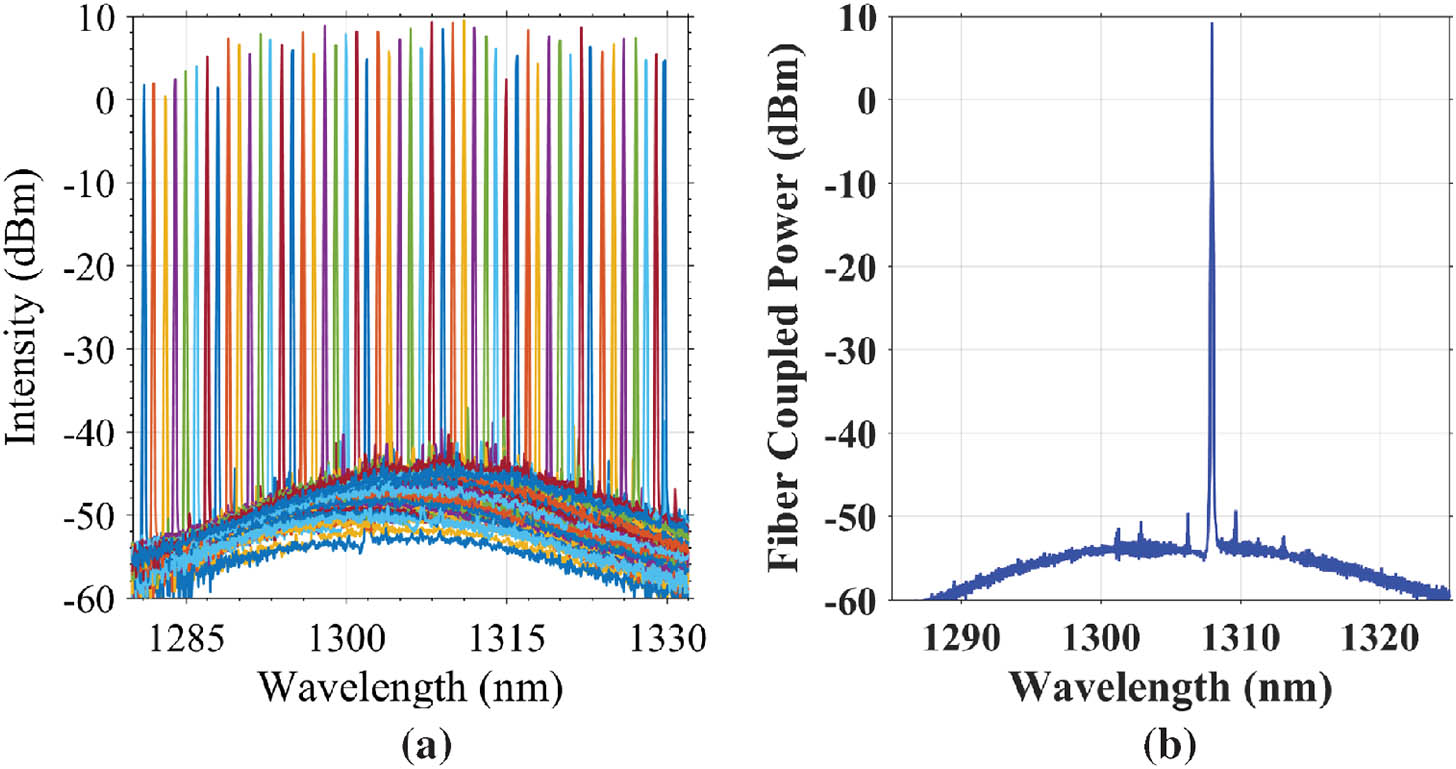
Fig. 7. Vernier ring MZI laser: (a) automatically measured spectra over the entire tuning range and (b) manually optimized spectrum at a single point showing 58 dB SMSR.
The measured tuning range and the SMSR for both devices decrease because of the gap reduction between the waveguide and the ring. With the correct design, the tuning range should increase to 70 nm for the Vernier ring MZI laser. Additionally, the gap reduction would increase the ring-bus coupling strength and, therefore, decrease the SMSR and increase the linewidth.
3.4 C. Spectral Linewidth
Linewidth enhancement in semiconductor lasers is a direct effect of coupling between the real and imaginary parts of the refractive index. This happens because any change in carrier density changes both material gain and the real part of refractive index. As a result, the effective linewidth is broadened from the predicted Schawlow–Townes linewidth. The linewidth for semiconductor lasers then becomes where is the modified Schawlow–Townes linewidth. The low loss passive cavity is beneficial in reducing the spectral linewidth of the laser. A detailed description on linewidth narrowing in heterogeneously integrated lasers is provided in Ref. [31]. Under external feedback, the linewidth of a laser is modified to where is the linewidth reduction factor and defined as The factors and are defined as where is the photon round-trip time in the active region, is the effective phase of the external cavity, and is the effective reflectivity of the passive section. The factor is proportional to the effective cavity length, which is increased by the photon re-circulation inside the ring resonators in the cavity. Since the volume of the active layer remains the same (fixed by the gain section length), the photons occupy a much larger volume when the effective cavity length increases thus reducing the net confinement factor. The decrease in confinement factor reduces the noise associated with spontaneous emission thus narrowing the linewidth. On the other hand, the factor is associated with optical negative feedback, which narrows the linewidth when lasing is red-detuned from the resonance of the mirror’s reflectivity.
To qualitatively understand the linewidth narrowing in Vernier ring lasers, we show in Fig.

Fig. 8. (a) Coefficients , , calculated for the Vernier ring laser cavity as functions of detuning from the ring resonance peak with . (b) Estimated Lorentzian linewidth as a function of linewidth enhancement factor. A waveguide loss of 5 dB/cm and an output power of 10 mW were used in the calculation.
Experimental study of the negative optical feedback in our laser’s linewidth is, unfortunately, outside the scope of this work due to equipment limitations. Linewidth, therefore, was measured when the lasing is tuned to be right at the resonance peak by maximizing the photocurrent in the on-chip PD. The linewidth for both lasers was measured at various spectral points across the wavelength tuning range. The measured Lorentzian linewidth as a function of wavelength is shown in Figs.

Fig. 9. Measured Lorentzian linewidth as a function of wavelength for (a) Vernier ring laser and (b) Vernier ring MZI laser.
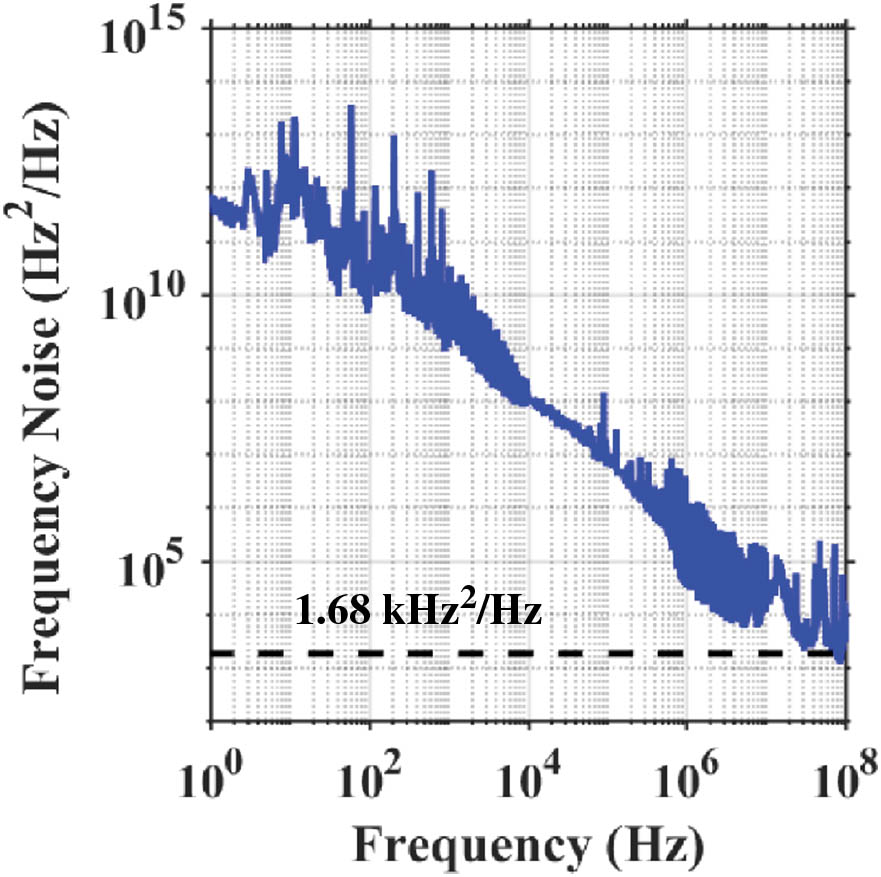
Fig. 10. Measured frequency noise of the Vernier ring MZI laser in logarithmic scale over complete frequency range.
4. CONCLUSIONS
This paper reports design and measurement results of widely tunable heterogeneously integrated QD lasers on SOI waveguides operating in the O-band. We show a wide tuning range and the lowest linewidths reported in the literature for integrated lasers operating in the O-band. After adjusting for grating coupler loss, we have power in the silicon waveguide. Further improvements can be made to increase both the tuning range and reduce the linewidth by including three or four rings in the passive cavity [23,31] or using a combination of rings and Bragg gratings [35]. A low-loss silicon waveguide platform with extremely shallow etched waveguides can further reduce side-wall scattering loss in the passive cavity [36] and lower the linewidth significantly. The QD gain material can itself be changed to reduce the linewidth enhancement factor by techniques such as -modulation doping. Even though these are the first demonstrations of heterogeneously integrated QD tunable lasers on silicon, the output power levels and the linewidth of our lasers are within the parameter range to be inserted in long-haul coherent communication networks.
5 Acknowledgment
Acknowledgment. The views and conclusions in this paper are those of the authors and should not be interpreted as presenting the official policies or positions, either expressed or implied, of DARPA, ARPA-E, or the U.S. government. The U.S. government is authorized to reproduce and distribute reprints for government purposes notwithstanding any copyright notation hereon.
Article Outline
Aditya Malik, Joel Guo, Minh A. Tran, Geza Kurczveil, Di Liang, John E. Bowers. Widely tunable, heterogeneously integrated quantum-dot O-band lasers on silicon[J]. Photonics Research, 2020, 8(10): 10001551.