Wide-field in situ multiplexed Raman imaging with superresolution
1. INTRODUCTION
Optical microscopy plays an important role in biological research, and various microscopy approaches have been demonstrated that provide wide fields and high spatial resolution [1
Various wide-field approaches for dynamic Raman imaging have been proposed over the last few decades [6
In this work, we combined SIM with SERS nanoparticles to provide wide-field multiplexed imaging and achieved a high lateral resolution of 109 nm. As a benefit of the small excitation volumes of samples restricted close to the coverslip surface, the method reduces the out-of-focus signals and is highly suitable for surface imaging. The narrow emission profiles of the Raman signals are further scanned using combinable tunable filters to perform multiplexed imaging. The filter-based method is strongly compatible with conventional optical microscopy-based imaging systems. Surface biological imaging methods, and particularly time-lapse-based methods, will benefit from the technique presented here.
2. EXPERIMENTAL SETUP AND METHODS
The SIM employs spatially patterned light to illuminate a sample, to generate a moiré effect to encode undetectable high-frequency information to make such information detectable. A series of intermediate images can be captured by varying phases of the structured patterns. Then high- and low-frequency information is separated and shifted to the corresponding positions to reconstruct superresolution images [3]. Figure
A self-assembled central-wavelength tunable bandpass filter group that combines a tunable long-pass filter (TLP01-628, Semrock, U.S.) with a tunable short-pass filter (TSP01-628, Semrock, U.S.) is used to select the target Raman spectra. The orientation of the tunable filters is precisely controlled using two independent motorized rotation stages (PRM1Z8, Thorlabs, U.S.). The central wavelengths and bandwidths of the assembled bandpass filters are calibrated using a spectrometer (QE65000, Ocean Optics, U.S.). In the experiments, the full width at half-maximum (FWHM) of the bandwidth of the assembled bandpass filters is
The weak inherent scattering signal is an unavoidable disadvantage in Raman imaging; however, the metal nanoparticles enhance the Raman scattering signals effectively. In this case, metal composite nanoparticles with diameters of approximately 46–50 nm composed of a gold core with a silver outer shell were used. To achieve multiplexed imaging, these SERS nanoparticles were then encoded using sorted Raman molecules: 4-mercaptophenylacetic acid (4-ATP) (Au/Ag/4-ATP, nanoparticle size of
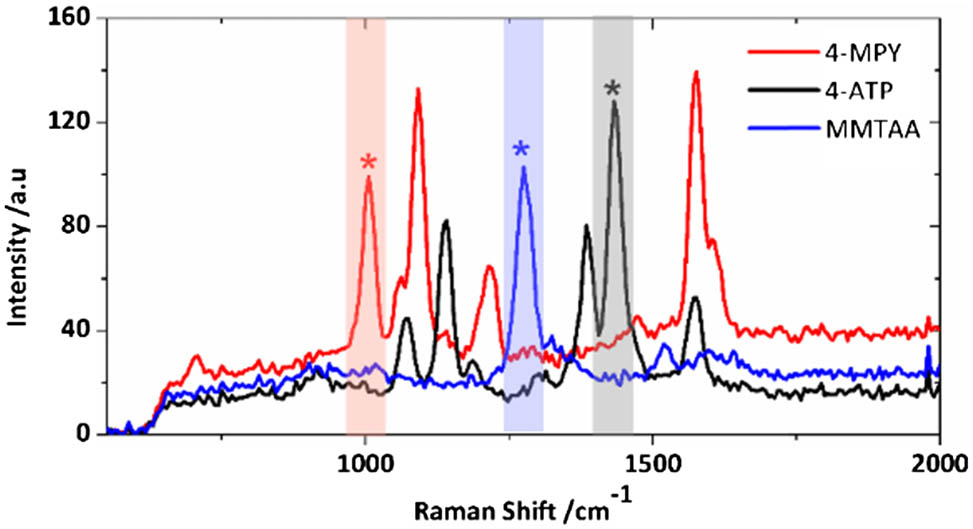
Fig. 2. Typical Raman spectra of the three different encoded SERS nanoparticles. Asterisks (*) indicate the selected peaks, and colored rectangular areas indicate the spectral regions that were used for multiplexed imaging in the experiments.
To perform multiplexed cellular imaging, the SERS nanoparticles were conjugated with the target molecules through an EDC (1-ethyl-3-[3-dimethylaminopropyl] carbodiimide hydrochloride)-NHS (N-hydroxysuccinimide) reaction [22,23]. The nanoparticles were modified using polyallylamine (PAH) by adding 250 μL of 10 mg/mL PAH solution to a 1 mL SERS nanoparticle solution overnight at room temperature under gentle shaking conditions. After PAH modification, the nanoparticles were then conjugated with the targeted molecules [the nuclear-targeting peptide (NLS), the mitochondria-targeting peptide (MLS) and the microtube-targeting molecule alpha-Tubulin DM1A]. Small amounts of the targeted molecule solutions [NLS (100 μL, 5 μg/mL), MLS (100 μL, 5 μg/mL), and DM1A (10 μL)] were mixed with a 100 μL 10 mM EDC solution and a 20 μL 100 mM NHS solution (1 mM = 1 mmol/L). These mixtures were then incubated at room temperature for 24 h under gentle shaking conditions. Finally, the nanoparticles were centrifuged and dispersed in a culture medium solution at 4°C for experimental use.
For the intracellular Raman imaging, 3T3 cells were first cultured on coverslips for a minimum of 8 h to allow the cells to adhere to the coverslip surfaces. Then, 5 μL of the modified SERS nanoparticle solution was added to each of the culture media simultaneously, and the cells were cultured further for another 24 h to phagocytize the nanoparticles. Before the imaging experiments, the cells were washed for three times using phosphate buffer saline (PBS). Additionally, to bring more of the targeted cell structures into the illumination volume, the cells were mechanically flattened using a 1-mm-thick piece of agarose gel (∼1.5 wt. %), and the agarose gel pad was then covered using a
3. RESULTS AND DISCUSSION
3.1 A. Stability of Raman Scattering
Photobleaching is an unavoidable problem in fluorescent imaging because of the long acquisition times or high excitation powers that are usually required. To calibrate the photostability, we measured and compared the scattering intensities of the fluorescent microspheres (diameter

Fig. 3. (a) Normalized intensities of fluorescent scattering and Raman scattering signals over time. The signals were detected at a frame rate of 0.2 Hz over an exposure time of 0.3 s. The red curve represents the Raman signal, and the black curve represents the fluorescent signal. (b) and (c) show time-lapse images of the fluorescent microspheres and the SERS nanoparticles, respectively.
3.2 B. Wide-Field Multiplexed Imaging of SERS Nanoparticles
The lateral resolution is of vital importance for wide-field multiplexed imaging systems. Therefore, for evaluation, three spectrally distinct SERS nanoparticles were initially mixed and imaged. The mixed solution of Raman molecule-encoded SERS nanoparticles was deposited on a coverslip surface and dried in the atmosphere. During the experiments, the nanoparticles were immersed in water to mimic an intracellular fluid environment. The structured illumination was implemented in two perpendicular directions, and six intermediate images were captured for each superresolution image reconstruction. This capture process was repeated for every bandpass filter. Figure
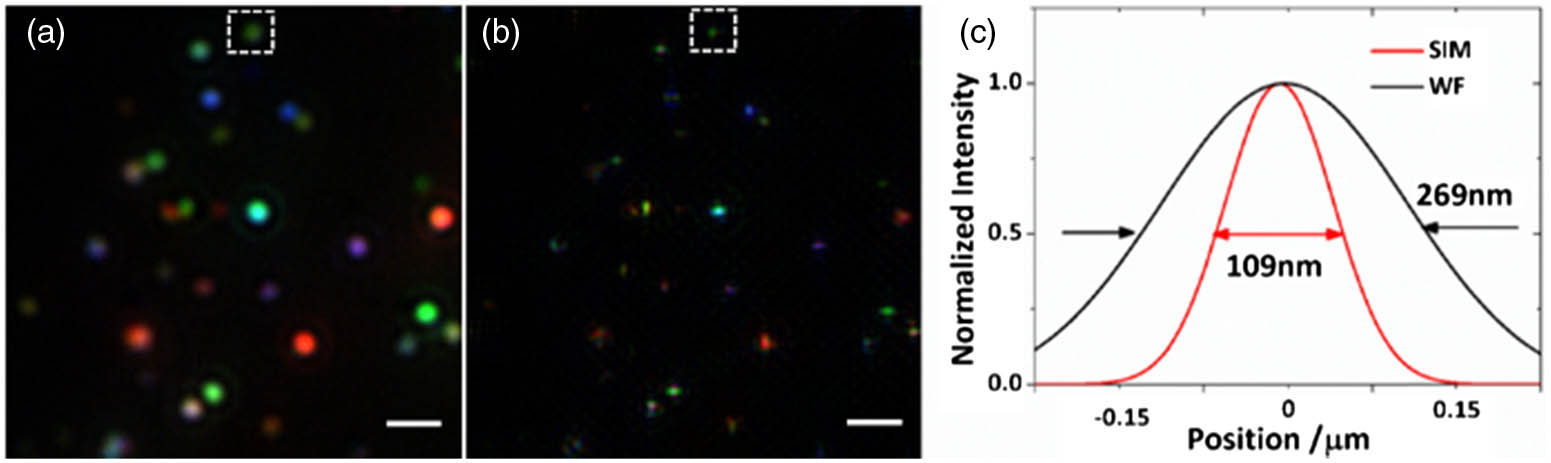
Fig. 4. Wide-field imaging of the SERS nanoparticles. (a) Conventional imaging results and (b) the reconstructed wide-field image; (c) Gaussian fitting profiles of the normalized intensity of the images framed in (a) and (b). Scale bar: 1 μm.
In these images, the primary colors are not very pure for two main reasons. First, aggregation of different SERS nanoparticles is uncontrollable, and the separation distance may be smaller than the resolution of the system, so it is difficult to distinguish the overlap among different adjacent nanoparticles. Second, Raman signals of different molecules in the junction regions between adjacent nanoparticles are simultaneously enhanced to be detected; this is known as the dimer enhancement [25]. However, efficiency of the dimer enhancement is closely related to the polarization direction of the illumination light. Consequently, the isotropy of the spatial resolution of these reconstructed wide-field images can be seriously affected by variable polarization directions [10]. Therefore, the polarization direction is kept unchanged at an angle of 45° with respect to the horizontal directions during the experiments, and its enhancement effects on the different directions of illumination are essentially suppressed in this work.
3.3 C. Multiplexed Imaging of 3T3 Cells
On this basis, we further implemented such a conceptual multiplexed imaging technique for use in biological applications. Three organelles of 3T3 cells were labeled using surface-modified Raman molecule-encoded nanotags: the Au/Ag/4-MPY SERS nanoparticles labeled nucleus were imaged in the red layer, the Au/Ag/4-ATP SERS nanoparticles labeled microtubes were imaged in green, and the Au/Ag/MMTAA SERS nanoparticles labeled mitochondria were imaged in blue. Figure

Fig. 5. Multiplexed imaging of 3T3 cells labeled using surface-modified SERS nanoparticles. (a) Bright field image of 3T3 cell, and (b) conventional and (c) reconstructed wide-field pseudo-color images overlaid with the framed bright field image from (a). Scale bars: 5 μm in (a), and 1 μm in (b) and (c).
These results confirm that the wide-field Raman imaging method has a multiplexed imaging capability and that higher multiplexed images are feasible by adding appropriate Raman reporter molecules. However, the reconstructions in the cells still show imperfections when compared with those of the SERS nanoparticles shown in Fig.
3.4 D. Discussion
In the wide-field Raman experiments, SERS signals are all excited simultaneously using the same laser, avoiding the need for different sources and thus helping to simplify the illumination system and reduce the system cost to a large extent. For most imaging techniques, the resolution (both spectral and spatial resolutions) always conflict with imaging time, and a balance between resolution and imaging speed is necessary. The proposed method is thus a great candidate method for such cases. It can essentially provide the high imaging speeds of the wide-field method and a relatively high spatial resolution beyond the diffraction limit simultaneously. The spectral resolution of
4. CONCLUSIONS
In summary, we have demonstrated a new concept for a wide-field multiplexed imaging technique that combines SIM and optically encoded SERS nanoparticle labeling. The method provides high spatial resolution that is far beyond the diffraction limit in a wide field. Wide-field multiplexed imaging could also be achieved through simultaneous using of multiple labels. With functionalized SERS nanoparticles, multiple specific structures in cells can be labeled and imaged simultaneously. Though the method is still in its preliminary stage of development, it shows powerful potential in biological applications, particularly for time-lapse imaging of living samples. It is expected to be beneficial for research in biology, medicine, and other related fields.
5 Acknowledgment
Acknowledgment. The authors gratefully thank Prof. Zhuyuan Wang and Shenfei Zong for providing the SERS nanoparticles.
Article Outline
Houkai Chen, Xiaojing Wu, Yuquan Zhang, Yong Yang, Changjun Min, Siwei Zhu, Xiaocong Yuan, Qiaoliang Bao, Jing Bu. Wide-field in situ multiplexed Raman imaging with superresolution[J]. Photonics Research, 2018, 6(6): 06000530.