In-fiber integrated optics: an emerging photonics integration technology [Invited]
Download: 930次
1. INTRODUCTION
Integrated technology plays an increasingly important role in the field of micro and nano optics and photonics. Integrated optics began with the concept proposed by Dr. Miller of Bell Laboratory in 1969[1]. It presents a prototype for integrated optics with dielectric materials as a substrate. In 1972, Somekh and Yariv proposed the idea of integrating optical devices and electronic devices simultaneously on the same semiconductor substrate[2]. Since then, researchers have made use of various materials and different fabrication methods to make integrated optical devices and photoelectric hybrid integrated devices.
Based on the concept of integrated optics, the fiber Bragg grating was proposed by Hill
Different from long distance optical fiber communication, in most cases, in-fiber integrated optics focuses on the interaction between the short-distance waveguide itself and its surrounding waveguides. At the same time, special attention is paid to the interaction between the optical fiber internal waveguide and the material outside the fiber. These interactions are often caused by the interaction between the optical fiber end or the evanescent light field of the fiber and surrounding matter. In some special cases, in-fiber integration optics is also concerned with the long-range transmission characteristics and their interactions with the surrounding environment. Such as, the application of multicore optical fiber-based space division multiplexing optical communication, or remote dual optical path distributed interferometry. In-fiber integrated optics is committed to achieving such a goal and is devoted to the following basic research: (1) design and manufacture new functional fiber; (2) develop integration technology of functional optical devices or components; and (3) further develop the multiple functional microsystem integration technology and method of in-fiber integrated optics. The aim is to complete and implement the tasks of light wave control, light wave signal exchange, information processing in optical fiber, and information extraction from the interaction between fiber and the surrounding environment.
2. FUNCTIONAL FIBERS FOR IN-FIBER INTEGRATED OPTICS INFRASTRUCTURE
We know that special microstructure waveguide core fiber is the basic substrate material for fiber integrated optics. It is the key to constructing fiber integrated optical devices and it is also the premise for realizing fiber integrated micro-optical systems. Based on the actual demand, the microstructure waveguide core of the optical fiber is investigated and reconstructed. For example, the twin-core polarization-maintaining optical fiber is needed for the development of integrated interferometers; the refractive index of each core slightly different from multicore optical fiber is required for integrated parallel multifiber Bragg grating; and multicore rare earth-doped optical fiber could be used to build multiwavelength integrated fiber lasers in parallel. Functional fibers could provide basic material assurance for the research of in-fiber integrated optics.
2.2 A. Optical Path Separation Multicore Fiber
Multicore optical fiber (MCF) is a typical refractive index guiding microstructured optical fiber. Multiple fiber cores in single fiber make it possible to construct more complex optical paths in a single fiber. Compared with single core single-mode fiber (SMF), it consists of multiple independent fiber cores (at least two cores) in one public cladding. Each core in the optical fiber is an independent light wave channel; therefore, multicore fiber is considered as a multilight-wave channel or multi-optical-path integrated optical fiber. Figures
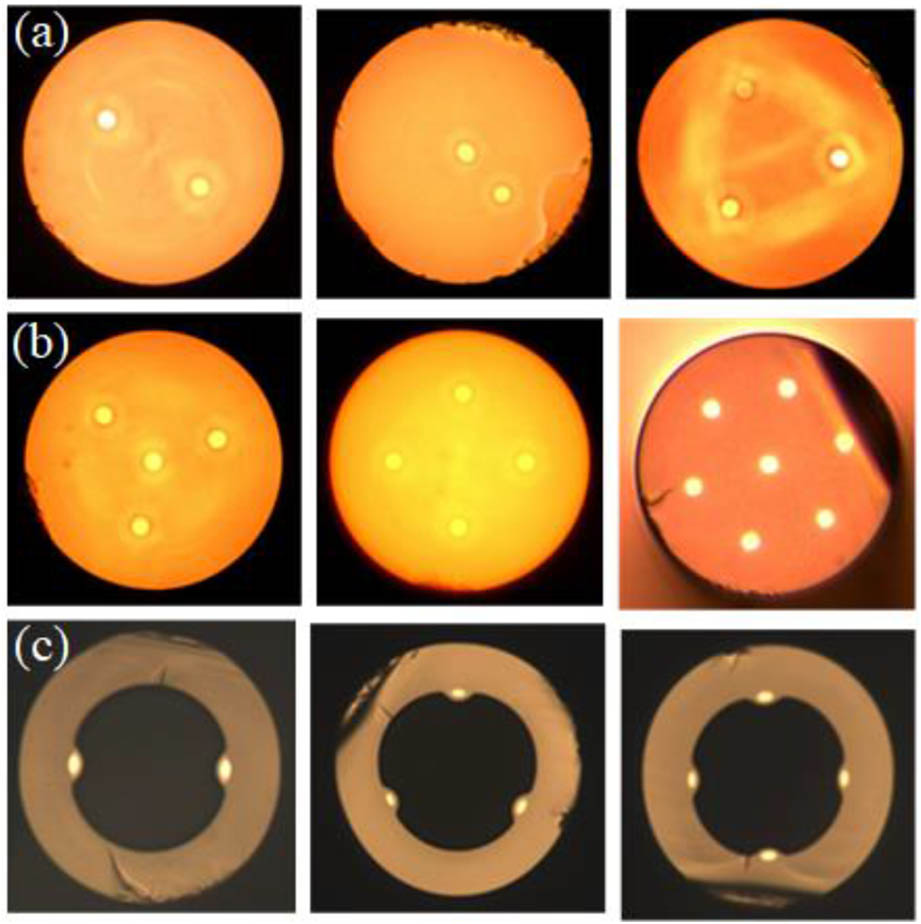
Fig. 1. Optical path separated multi-core fibers. (a) Twin-core and three-core fibers. (b) Four-core and seven-core fibers. (c) Central holey multi-elliptic-core fibers.
2.3 B. Multicore Optical Fiber for Light Beam Control
In a discrete optical system, waveguide arrays can be used to control the optical wavefronts with desired diffraction properties[14]. If multiple cores are arranged in a well-designed array, the control of the light field can be realized through the mutual coupling between the waveguides. Through the coupling between adjacent cores, the input light can broaden the spatial distribution[1517" target="_self" style="display: inline;">–
2.4 C. Optical Paths and Microfluidic Materials Channel Hybrid Integrated Fiber
If microfluidic channels and optical waveguides can be integrated into a single optical fiber, they will have great potential in optofluidic devices. They can realize convenient optical coupling and obviously increase the integration level of the devices. Microstructured optical fibers with microholes in the micrometer scale diameters can contain gas or liquid with the volume of a microliter or a nanoliter. Therefore, they are desired carriers for trace detection. This one-dimensional holey structure provides a long cell for the contact between samples and the waveguide, which breaks through the limitations of the traditional optical fiber and exhibits irreplaceable advantages in the fields of analyses. Here, a series of specially designed optical fiber is given in Fig.
2.5 D. Photonic Crystal Fibers
The photonic crystal fiber (PCF), owing to the arrayed microhole structure, makes it suitable for biochemistry sensing, strain and temperature measurement, and numerous optical integrated devices. The PCF with a solid core can be filled with liquid or polymers into the surrounding holes for evanescent-wave sensing of chemicals or biomolecules, as in Figs.
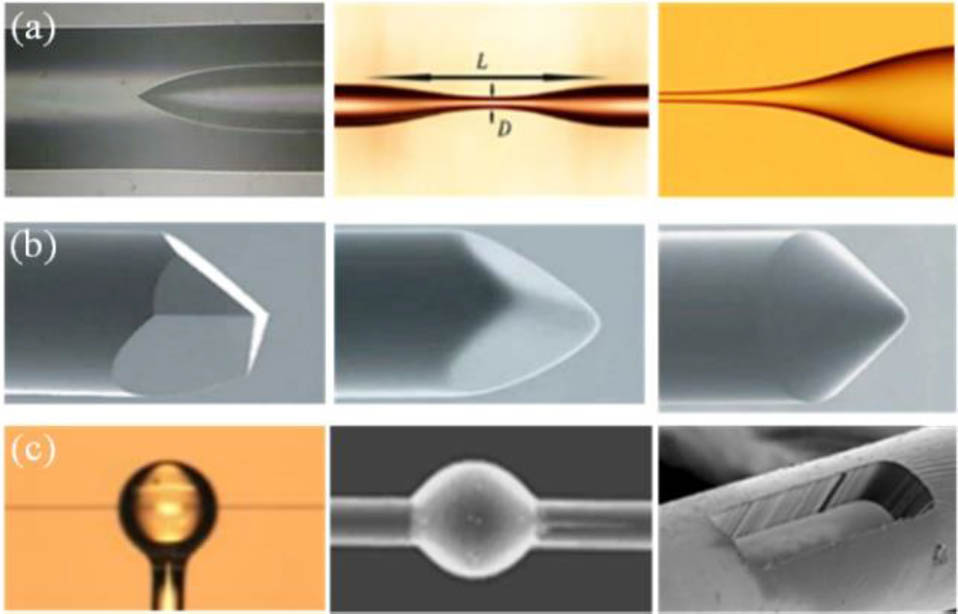
Fig. 5. In-fiber integrated optical components based on several fabrication techniques. (a) Fused splicing and tapering of fiber components. (b) Optical fiber end components by polishing. (c) Fused sphere, holey fiber bubble by laser and groove by 157 nm laser.
3. FUNDAMENTAL OPTICAL DEVICE CONSTRUCTION AND FABRICATION
Based on the fibers mentioned above, many micro processing technologies, including fused splicing technology for in-fiber coupler/splitter, the side polishing technique, fiber end machining, melting and tapering, chemical etching and coating, and laser micro mashing technology[22], were introduced to fabricate several in-fiber integrated optical devices and components[15,23
3.2 A. In-fiber Integrated Coupler
There are two ways to build an in-fiber integrated coupler for multicore fibers. One is to realize the coupling of the light wave by fused splicing of the SMF with the multicore fiber, and then heating and stretching at the splicing joint to make a bi-tapered fiber zone, thus light waves could be coupled into each core of the multicore fiber from the SMF[24,29], as shown in Fig.
3.3 B. Multicore Fiber Fan-out Device
In transmission systems, one of the key components used to realize space division multiplexing (SDM) and expand the transmission capacity is the multicore fiber fan-out component as a connecting device between the multicore fiber and SMF[13]. A “vanishing core” technology[32] can be used to fabricate the multicore fiber fan-out component. A four-core fiber fan-out device, as an example, is fabricated by four double cladding fibers embedded in a fiber substrate, as shown in Fig.
3.4 C. Multicore Fiber Bragg Grating
Multicore fiber can be used to write Bragg gratings in parallel. Due to the multiple parallel fiber grating can not only be used to measure the tensile strain, but also can be used to measure the differential strain. Therefore, this kind of fiber Bragg grating integration device is useful for configuring a fiber optic bending sensor for curvature measurement[33]. In the writing process of multicore fiber Bragg gratings, because the fiber core deviates from the axisymmetric center, the fiber itself as a cylindrical lens has a certain effect on the quality of Bragg gratings writing. Fig.
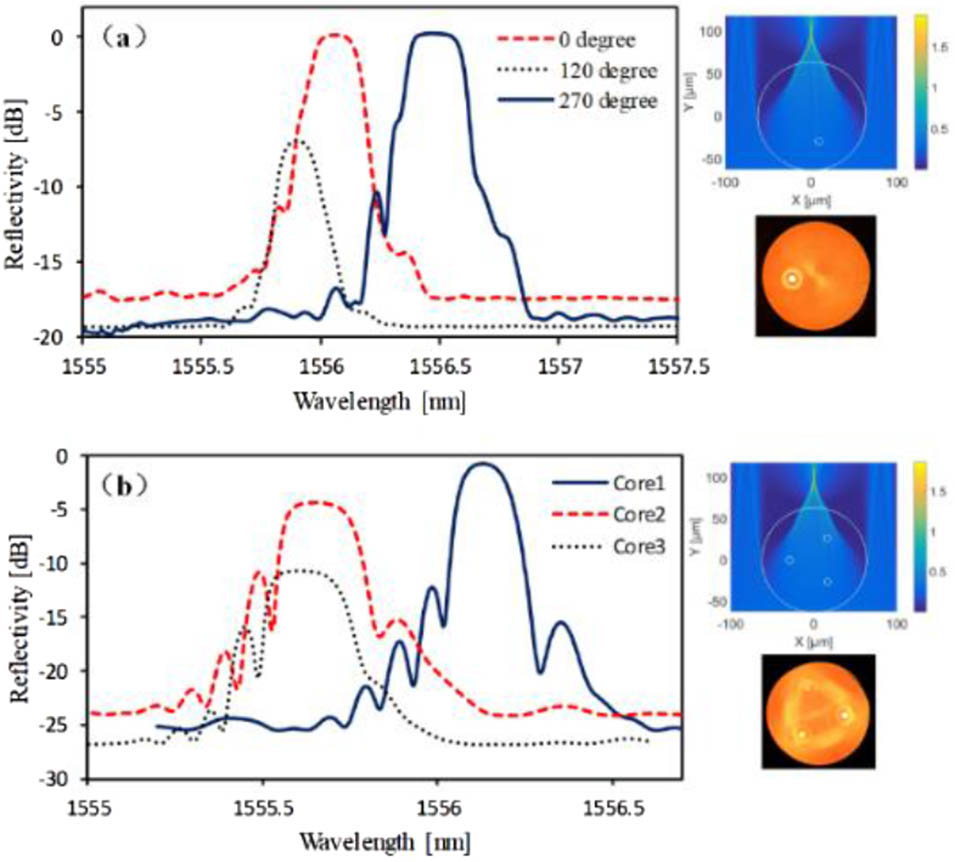
Fig. 8. Eccentric core fiber and three-core fiber Bragg gratings written by a phase mask.
3.5 D. In-fiber Integrated Modulator
Poled fiber can be constructed as an in-fiber integrated modulator for communication applications, especially an intrinsic phase-modulating device, including electro-optic intensity modulators, and Mach–Zehnder or other types of interferometers[35].
By using the special designed hollow twin-core fiber, in which the cores have an elliptical shape and are embedded in the inner wall of the silica capillary tube, as shown in Fig.
3.6 E. In-fiber Integrated Multi-lasers
As the multicore fiber can carry or transmit information in each core separately, the fiber amplifications or the fiber lasers can also be integrated into multicore fiber to form a multi-laser, which can substantially lower the cost and reduce the size, such as seven-core fiber distributed feedback (DFB) lasers[36]. In the implementation process, multicore fiber

Fig. 10. Seven-core fiber multi-laser and experimental setup. (a) Lateral UV beams on seven-core fiber. (b) Ray trajectory of UV writing beam exposing all cores without obstruction. (c) Transmission spectrum of each core measured by a scanned laser. (d) Experimental setup for measuring the MC-DFB.
3.7 F. In-fiber Integrated Photodetector
Integrating optical fiber with optoelectronic materials is meaningful work. The incorporation of semiconductor junctions into microstructured optical fiber can effectively improve the performance of in-fiber devices or all-fiber optical devices, enabling generating, modulating, and detecting light within the fiber itself (Fig.
The high-pressure chemical vapor deposition (HPCVD) technology can implement the fiber-compatible optoelectronic device fabrication[37]. The process of integrating needs chemical precursor mixtures flowing through microholes by high pressure, precursor decomposition, film deposition, and dopant incorporation. By varying the pressure and controlling the concentration of the dopant, different layer thickness can be achieved. The holey structure is facile to construct semiconductor optoelectronic junction inside the hole. As the semiconductor layers are parallel to the fiber core, it is applicable to efficiently and directly couple light from the fiber core into the semiconductor layers. In the transverse direction of the fiber mode interaction and power transfer can couple from the core into the junctions. Thus, the in-fiber integrated optoelectronic device can realize many functions as transistors, light-emitting diodes, and photodetectors.
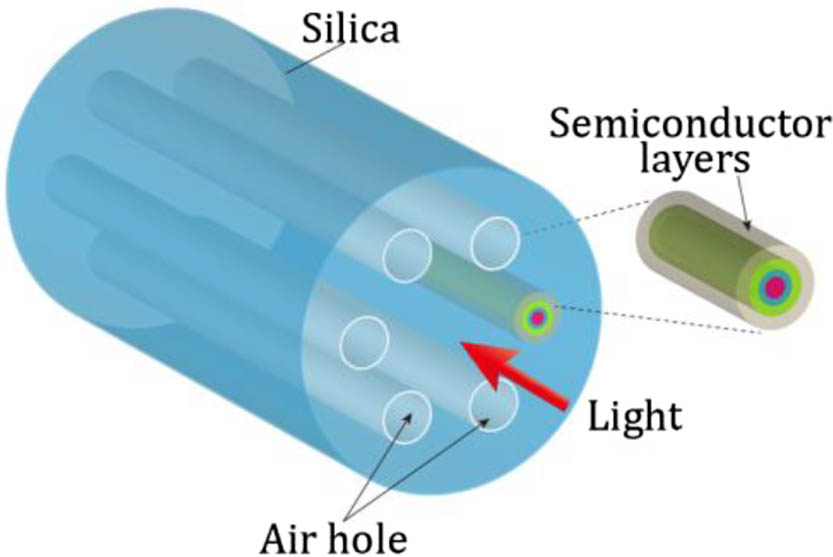
Fig. 11. Optoelectronic photonic crystal fiber with a coaxial semiconductor junction. The junction is composed of several layers of silicon and germanium and is located in a hole adjacent to the fiber core.
4. EXAMPLES OF IN-FIBER INTEGRATED OPTIC SYSTEM
Several functional optical devices are integrated into the same cylindrical optical fiber substrate, which can form a fully functional micro in-fiber integrated optical system. Therefore, it is of great significance to promote the application of in-fiber integrated devices from a single functional unit to multifunctional in-fiber integrated optical microsystems. In order to illustrate the potential applications of in-fiber integrated optics technology, we give some examples, such as a microfluidic device integrated into one fiber constituting a microstructure chemical sensing or microanalysis system; an in-fiber integrated interferometer forms a single fiber-based micro accelerometer; and by using a twin-core fiber and fiber end micro processing technology, an optical fiber-based micro particle manipulation system is also configured.
4.2 A. In-fiber Integrated Optofluidic System
In-fiber integrated optofluidic devices have been used in the fields of chemical and environmental analysis, biosynthesis, and drug delivery due to their specific advantages such as microfluidic control, a large specific surface area, and an integrated light path[3840" target="_self" style="display: inline;">–
Specifically, they chose hollow suspension core fibers for experiments, as shown in Figs.
4.3 B. In-fiber Integrated Accelerometer
The twin-core fiber can be used to construct a variety of in-fiber integrated devices, such as Michelson interferometer[21] and micro accelerometer[41].
In the micro accelerometer measuring system, the sensing element is based on a twin-core fiber Michelson interferometer, as shown in Fig.
4.4 C. Particle Trapping Micro-optic System
The micro-optic integration system example for particle trapping and manipulating consists of twin-core fiber with an abruptly tapered tip, as shown in Fig.
4.5 D. In-hollow Core Photonic Bandgap Fiber Integrated Gas Sensing System
The hollow-core photonic bandgap fibers (HC-PBF), by virtue of the cellular structure, are especially suited for liquid or gas sensing[44]. The HC-PBF can simultaneously serve as light transmission cables and sensing elements with a long length. The experimental setup for detecting gas with HC-PBF is shown in Fig.
5. CONCLUSIONS
The refractive index microstructure waveguide fiber is a vital research field of fiber optics. Compared with traditional optical fiber, its structure is a big difference, with so many new functions and new features added. These features can be used not only for optical information processing and optical communication devices, but also can be used in the in-fiber integrated optics. The purpose of in-fiber integrated optics is to realize the miniaturization of fiber-based optical devices; it makes optical information transmission, sensing, exchanging, and processing devices more compact. This emerging photonics integration technology is evolving into a new branch of micro and nano photonics. The main concept of the in-fiber integrated optics is to integrate the optical path and various optical components into a single optical fiber. Here, we remark on the basic in-fiber integrated technology including multicore microstructured fiber, in-fiber integrated optical devices, and some application examples to explain this emerging photonics integration methodology. Varied applications can be found in micro/nano optics integration systems.
[1]
[2]
[3]
[5]
[6]
[7]
[8]
[9]
[10]
[11]
[12]
[13]
[14]
[15]
[16]
[17]
[18]
[19]
[20]
[21]
[22]
[23]
[24]
[25]
[26]
[27]
[28]
[29]
[30]
[31]
[32]
[33]
[34]
[35]
[36]
[37]
[38]
[39]
[40]
[41]
[42]
[43]
[44]
[45]
Article Outline
Xiaotong Zhang, Tingting Yuan, Xinghua Yang, Chunying Guan, Jun Yang, Zhihai Liu, Hongchang Deng, Libo Yuan. In-fiber integrated optics: an emerging photonics integration technology [Invited][J]. Chinese Optics Letters, 2018, 16(11): 110601.