High-sensitivity, high-spatial-resolution distributed strain sensing based on a poly(methyl methacrylate) chirped fiber Bragg grating
Download: 644次
1. INTRODUCTION
Optical-fiber-grating-based sensors are emerging as promising devices owing to their high stabilities, high reliabilities, and advanced multiplexing capabilities [1]. In recent years, the application of chirped fiber Bragg grating (CFBG) with a nonuniform modulation of the refractive index within the core of an optical fiber has attracted considerable attention [2].
For high-spatial-resolution strain-sensing applications, such as tactile perception and aircraft structural detection [3,4], CFBG behaving as a cascade of FBGs of different Bragg wavelengths is a promising candidate for fully distributed sensors owing to its large grating length, spatial dispersion characteristic, and large reflection bandwidth. The strain information is encoded into the wavelength-dependent reflection spectral range. To interrogate the reflection spectrum, an optical spectrum analyzer [5], Fabry–Perot filter [6], microwave photonic filter [7], and time-stretch frequency-domain reflectometry [8] have been used. However, the performances of these interrogation techniques are limited by either the lack of spatial resolution or high-speed data acquisition requirement. With the reasonable measurement time and considerably higher spatial resolution, optical frequency-domain reflectometry (OFDR) is a powerful method for the measurement of a small reflection signal in small-scale optical components [9]. The distributed strain information along the grating section of the CFBG can be reconstructed theoretically from the recorded temporal interference waveform and interrogated by means of OFDR in a Michelson interferometer. Therefore, it is possible to detect spatially resolved variations in strain with a resolution on the order of millimeters over the grating length.
In addition to the high spatial resolution, high sensitivity is another important factor for the distributed strain-sensing technology. In most cases, strain is due to the applied external force, so the strain sensing is actually a measurement of external force. According to Hooke’s law, polymer materials have higher sensitivity to converting external force into internal strain of the optical fiber, compared with silica material. Therefore, the mechanical properties provide increased sensitivities to intrinsic polymer fiber sensors when they are used for lateral force, stress, and torque sensing. Recently, polymer materials such as CYTOP, TOPAS, and PMMA have been researched for polymer optical fiber (POF) fabrication and application. Liu et al. [10] reported an axial tensile experiment using PMMA FBGs with a maximum strain of 3.61%. Leal et al. [11] measured the torque of an elastic actuator’s spring based on CYTOP FBG arrays in the strain range of thousands. Woyessa et al. reported a single-mode POF using ZEONEX/TOPAS for high-temperature sensing [12]. Leal et al. reported a diaphragm-embedded sensor applied on the pressure, force, and liquid level assessment using CYTOP fibers [13]. Among these materials, PMMA was the preferred material for producing POF because of its relative ease of fabrication, high optical transparency in the visible region, and refractive index similar to that of a conventional silica optical fiber [14]. CFBGs in PMMA-based optical fibers are promising sensing devices owing to their fundamental mechanical advantages over silica fibers (73 GPa) including a lower Young’s modulus (2.3 GPa) [15], higher elastic limit, simple installation in a narrow space, and improved biocompatibility for sensing applications [16]. Min et al. and Marques et al. have reported that the PMMA-based CFBG can be used as an average strain sensor with a good sensitivity [17,18].
In this study, distributed measurement of strains along the different stress points of a single PMMA CFBG is studied innovatively. We fabricate a single-mode PMMA POF using a unique core dopant, diphenyl disulfide (DPDS), providing both increase in core refractive index and photosensitivity. The fabrication method is reported in Ref. [19]. CFBGs are inscribed in the PMMA POF using a chirped phase mask. The ultraviolet (UV) light source is a 248 nm KrF excimer laser. Our aim is to study the distributed strain-sensing ability of this novel PMMA CFBG. Compared with silica CFBG in an experiment, PMMA CFBG shows that it could realize distributed strain sensing with a good agreement between the theory and the experiment. To the best of our knowledge, for the first time, we have achieved a high-spatial-resolution, high-sensitivity distributed strain-sensing based on a PMMA CFBG, with a spatial resolution of 1 mm over a gauge length of 40 mm and strain resolution of 0.491 Hz/με.
2. SCHEMATIC AND THEORY
Figure
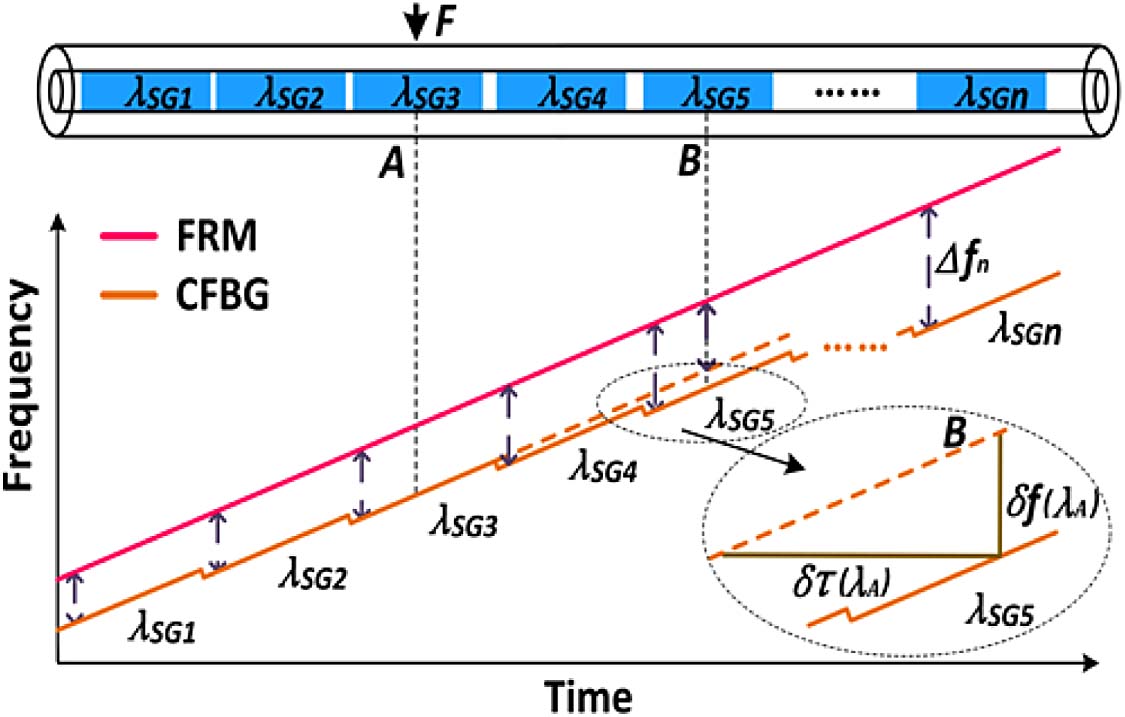
Fig. 1. Frequency relationship between the CFBG and FRM and corresponding beat frequencies. Inset: differential frequency offset of the CFBG under strain. SG, grating section.

Fig. 2. Schematic of the experimental setup. Inset: beat frequency relationship between the time and position. The blue dotted line is the linear fit, which reflects the distance-induced beat frequency, while the black spots show the DFO. SS, swept laser source; PD, photodiode; PA, power amplifier; DAQ, data acquisition; DSP, digital signal processor.
By beating the signals from the FRM and the PMMA CFBG at the photodetector (PD), beat signals with a microwave frequency dependent on the Bragg wavelength and proportional to the time-delay difference between the two reflected waveforms are generated, which could be used as a baseline to measure and locate the strain information along the PMMA CFBG: where is the sweep rate, α = , where is the sweep range and T is the sweep period; and is the distance-induced time delay , owing to the different positions of the FRM and PMMA CFBG grating section.
Therefore, the beat signals generated by the time-delay difference correspond to the chirped dispersion wavelength and spatial position of the PMMA CFBG as shown in Fig.
A lateral force applied at a certain position on the PMMA CFBG produces an axial stress [20] where is the Poisson’s ratio of PMMA of 0.4, is the lateral force zone, and is the diameter of the optical fiber. The strain at this position can be expressed as where is the Young’s modulus of PMMA. The change in local strain modifies the specific Bragg wavelength at the local position. Thus, the instantaneous Bragg wavelength shift at this position can be expressed as where is the wavelength shift induced by the strain, is the effective strain-optic coefficient of PMMA of 0.034, is the initial wavelength at a specific reflection position A in the PMMA CFBG, and is the applied strain.
For the PMMA CFBG with a chirp rate of [nanometers per centimeter (nm/cm)], by using Eq. (
3. EXPERIMENTS AND ANALYSIS
An experiment is performed to verify the utility of the proposed technique. Figure
To demonstrate the effectiveness and practicability of the proposed method, we experimentally compare the distributed strain-sensing abilities of silica CFBG and PMMA CFBG. According to the reflection bandwidths of both CFBGs, a swept laser source (Keysight 8164B) is adjusted and provides a linear wavelength modulation in the range of 1520–1620 nm with a sweep rate of 200 nm/s. The light wave from the swept laser source is sent to the FRM and CFBG through an isolator and a 3 dB fiber coupler with a weak wavelength-dependence loss. The short-wavelength end of the CFBG is connected to the coupler. The output of the interferometer with the wavelength-swept source produces beat signals at the PD (Thorlabs DET01CFC). The relative position of the FRM could be set before or after the CFBG according to the application, which only affects the increase or decrease in beat frequency in the same sweep direction, as shown by experiments. By adjusting the distance between the FRM and CFBG, the beat signals can be acquired by a low-speed data acquisition card (NI6010 200 kHz). A fully distributed strain along the CFBG could be measured through a time-/space-resolved short-term Fourier transform (STFT) analysis.
In the experiments, the resonance wavelength of the sensing silica-CFBG is centered at 1545 nm with a reflection bandwidth of 40 nm as shown in the upper inset of Fig.
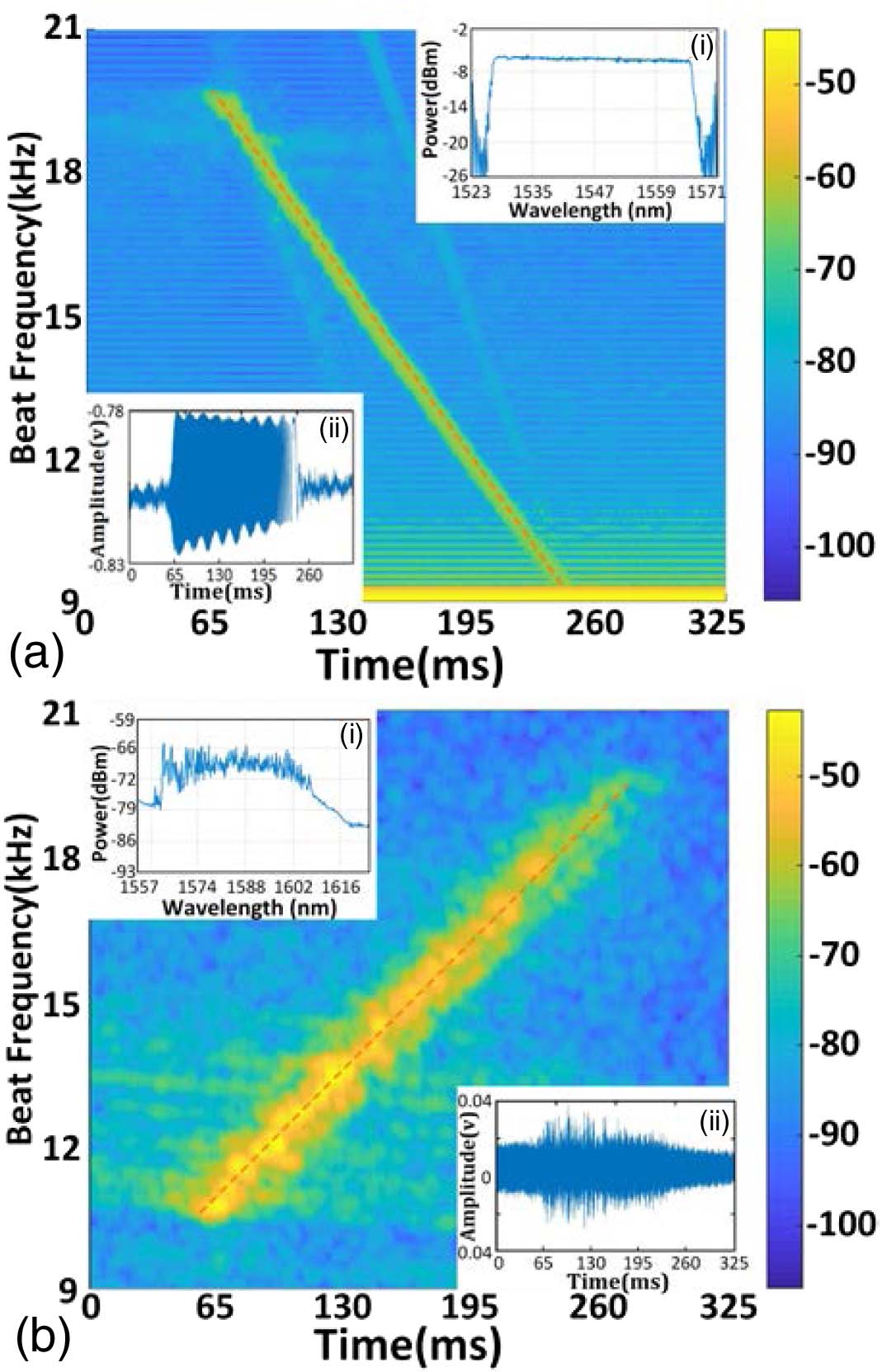
Fig. 4. Microwave spectrogram calculated by STFT without strain. (a) Silica fiber. (b) PMMA fiber. Insets: (i) measured reflection spectrum and (ii) measured initial temporal interference waveform.
When an external lateral force is applied to the two types of CFBGs at a certain carrying position, their strain responses are different, owing to the different characteristics of the two materials. As shown in Fig.
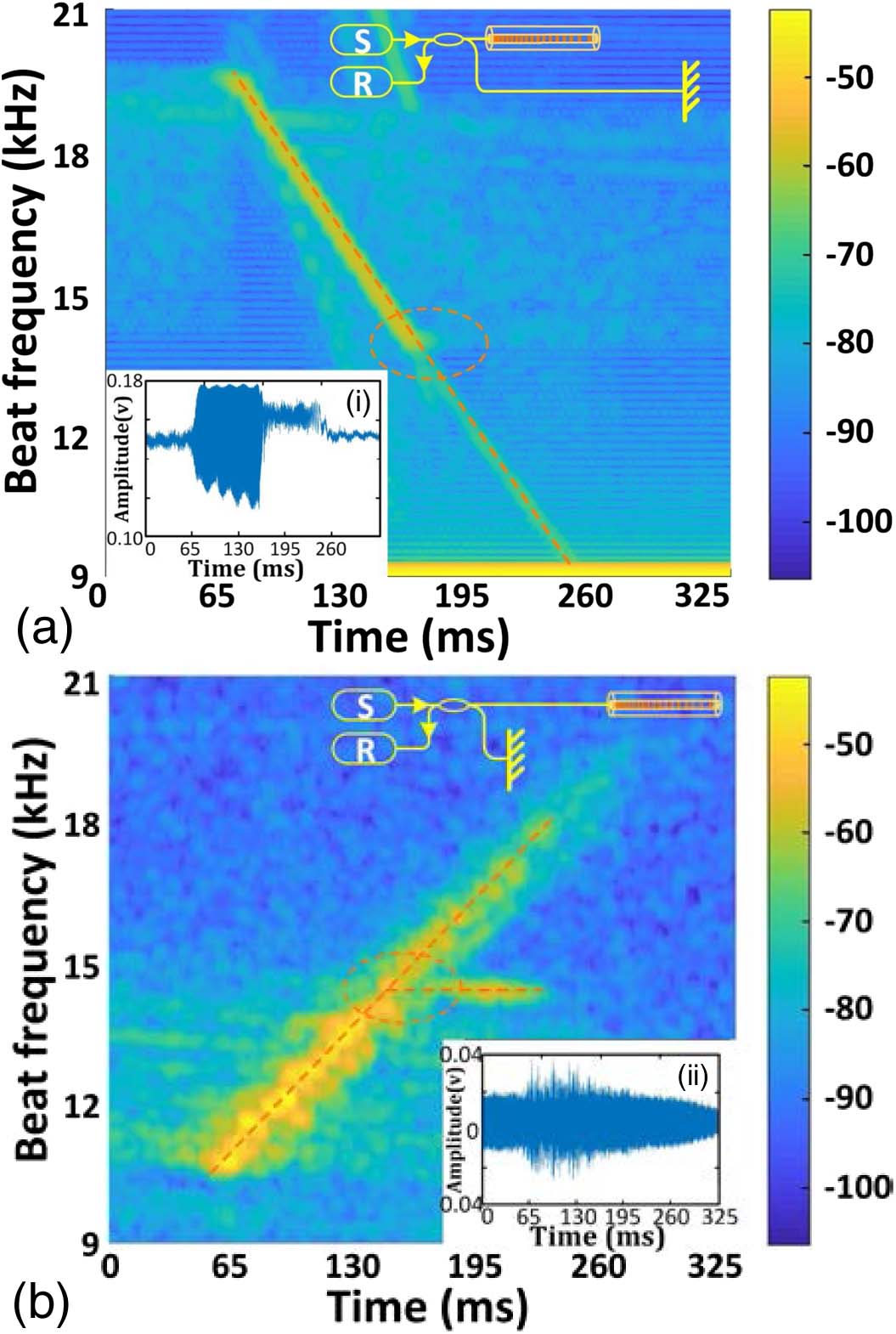
Fig. 5. Microwave spectrogram calculated by STFT under strain. (a) Silica fiber. (b) PMMA fiber. Insets: reflection spectra of the (i) silica and (ii) PMMA fibers under stress.
As shown in Fig.
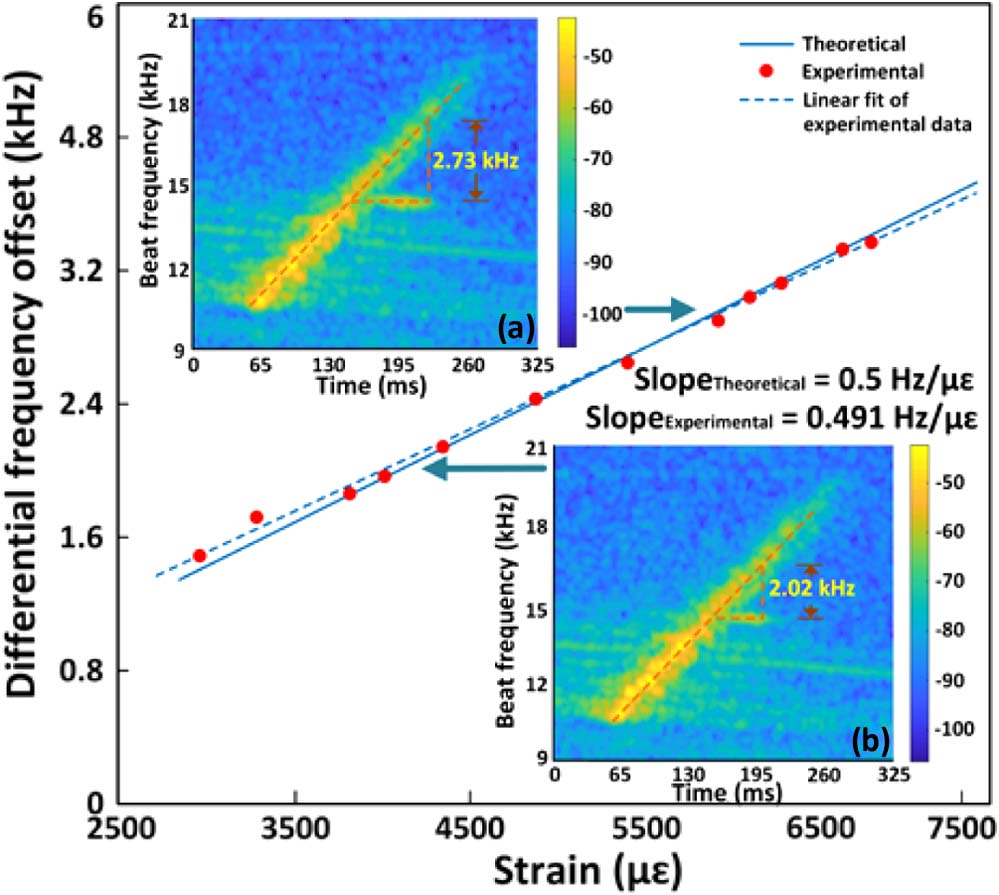
Fig. 6. DFO at various strains. The insets show spectrograms of the temporal interference patterns at uniform strains of 4060 and 5540 με.
Considering the high strain sensitivity and high spatial resolution of the proposed technique, we analyze the capability of the PMMA CFBG to identify different stresses at different positions of the sensor grating. We glue the sensing PMMA CFBG on top of a substrate and simultaneously load stresses at different positions. As shown in Fig.
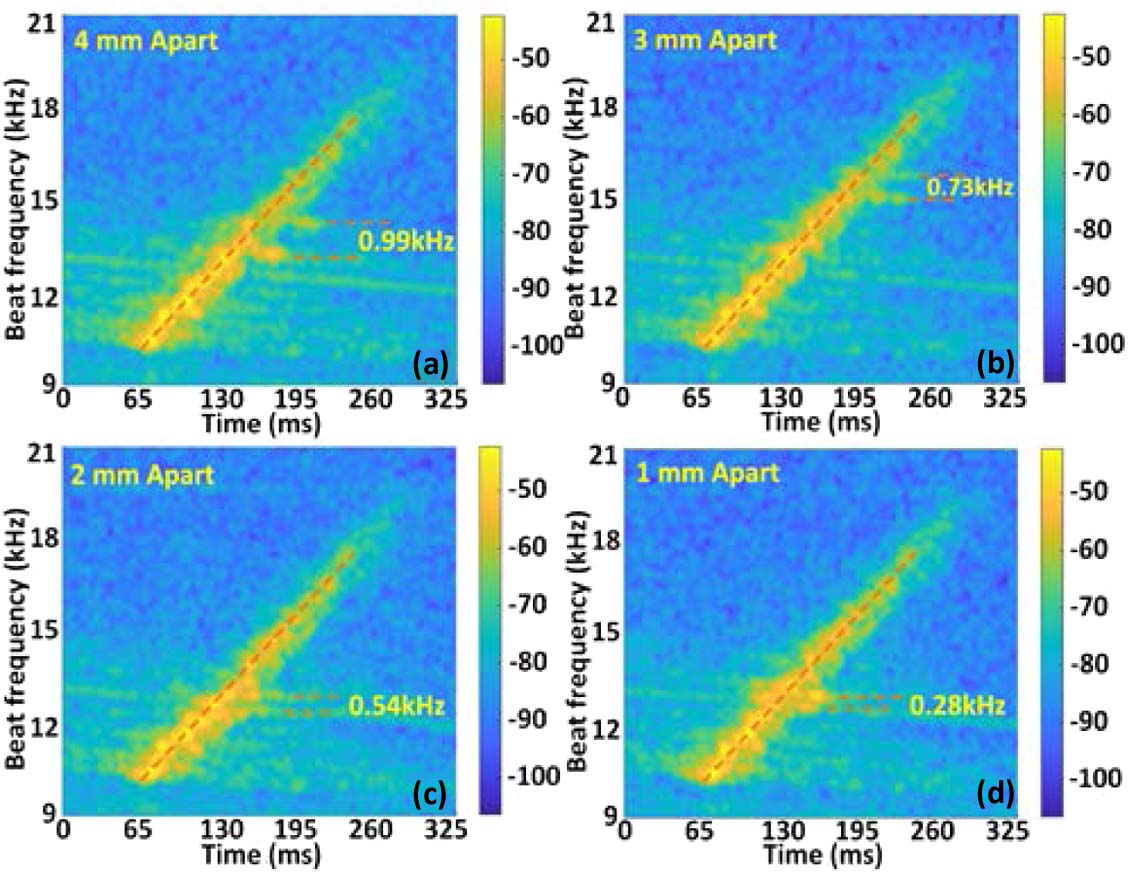
Fig. 7. Characterization of the system by applying various uniform strains at distances of (a) 4 mm, (b) 3 mm, (c) 2 mm, and (d) 1 mm.
Figures
As we know, the quality of fiber drawn from preform can affect the quality of CFBG. So we think that the improvement on the preform must be investigated from the two aspects: the uniformity of the dopant in the core area must be improved, and the surface of the preform after processing must be smoother. According to the aforementioned information, in the future, we may try adding no extra dopant (in this paper, DPDS is the extra dopant) [12]. Therefore, by improving the fabrication quality of PMMA CFBG devices, combined with the relevant filtering algorithm and other conditions to solve the SNR problems, it is believed that the spatial resolution ability is not limited to 1 mm with the method of this paper.
4. CONCLUSION
We propose and experimentally demonstrate a fully distributed strain-sensing approach using PMMA CFBG based on OFDR with high spatial resolution and sensitivity. The principle of the approach is to transform the distributed strain and location information along the grating length to the DFO through the distance-induced time delay and strain-induced dispersion time delay. The proposed technique is verified by experiments. A spatial resolution of 1 mm over a gauge length of 40 mm and a strain resolution of 0.491 Hz/με are achieved. One measurement cycle is about 200 ms according to the sweep rate of the light source in this paper. The spatial resolution depends on the reflection response ripples of the PMMA CFBG, while the strain resolution and demodulation speed depend on the chirp rate of the PMMA CFBG and sweep speed of the light source [21]. Considering the high sensitivity of the PMMA fiber, by improving the inscription quality of the CFBG and sweep rate of the light source and using a new material with a considerably lower Young’s modulus for the POF, the proposed technique could be a promising distributed strain-sensing approach for short-range fully distributed sensing systems where high spatial resolution, sensitivity, and measurement speed are required.
Chengang Lyu, Ziqi Liu, Ziqiang Huo, Chunfeng Ge, Xin Cheng, Haw-Yaw Tam. High-sensitivity, high-spatial-resolution distributed strain sensing based on a poly(methyl methacrylate) chirped fiber Bragg grating[J]. Photonics Research, 2020, 8(7): 07001134.