Chemistry under extreme conditions: Pressure evolution of chemical bonding and structure in dense solids
1 I. NOVEL STATES AND NEW MATERIALS UNDER EXTREME CONDITIONS
The materials that exist deep in planets and stars experience extremely high pressures (HPs) and temperatures (
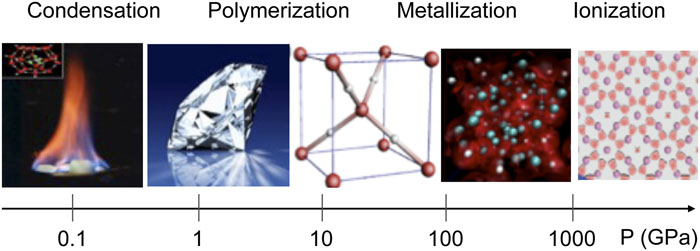
Fig. 1. Novel states of materials occur under a wide range of extreme pressures, which include methane hydrates at 0.1 GPa, diamond at 5 GPa, symmetric ice at 80 GPa, metallic hydrogen at 500 GPa, and Al electrides at 10 TPa. These pressures can be generated by modern high-pressure technologies in static and dynamic conditions (Fig. 2 ).
Materials under extreme conditions undergo significant changes in their bonding, structures, and properties that have significant implications for the fundamental sciences, materials, and technologies. For example, the compression energy at the bottom of the ocean (∼0.1 GPa) rivals the hydrogen bond energy that drives water to form clathrates with captured methane inside.
High-pressure (HP) research examines the novel states, transformations, and properties of dense elemental solids that may constitute the interiors of giant planets and exoplanets. HP research provides fundamental materials data over a large range of pressure, temperature, and compression energy, which is critical to development and validation of new condensed matter theories and planetary models. HP research also provides fundamental insights into the stability-structure-property relationships of solids, which is critical to the development of new materials and structures that are stable at or near ambient conditions. In recent years, there has been an unprecedented number of discoveries of new materials and material structures, establishing pressure as a new dimension in materials research.
In this paper, we present several fundamental concepts governing the chemistry of dense solids under extreme conditions, retrieved from the recent discoveries of new materials under extreme conditions. The focus will be on understanding the pressure-induced evolution of chemical bonding and structure in dense solids, from molecular to covalent, ionic and, ultimately, metallic solids under extreme conditions. We also focus on the novel properties and complex transition mechanisms arising from the subtle balance between electron hybridization (bonding) and electrostatic interaction (packing) in densely packed solids.
This paper is organized as follows: We first describe modern high-pressure experimental methods in Sec.
2 II. EXPERIMENTAL METHODS
Studies of highly compressible molecular solids under extreme conditions are very challenging for several reasons. These include: (i) the difficulties in achieving extremely high pressure-temperature (PT) conditions; (ii) the absence of an in situ structural probe in a small number of samples, inevitable at high static pressures; and (iii) the transient nature (sub-nanosecond) of the compressed states encountered at high dynamic pressures (
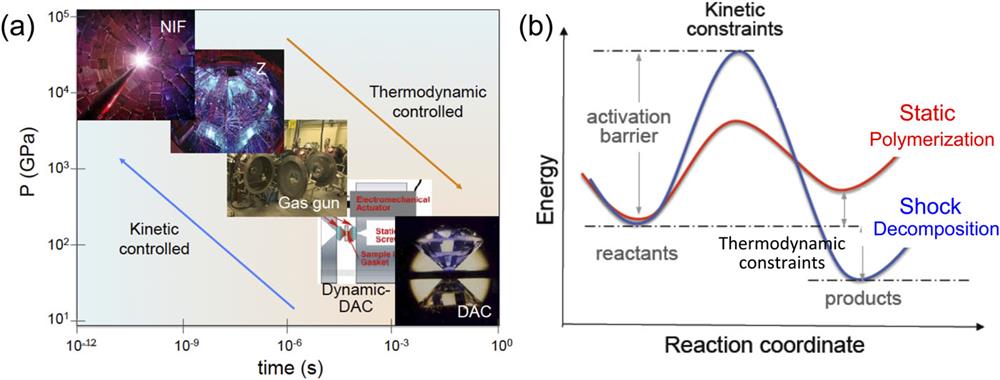
Fig. 2. (a) Modern high-pressure technologies, such as DAC, dynamic -DAC, gas guns, Z-machine, and NIF are capable of generating the extreme PT conditions in the deep interiors of the giant planets at different time scales. (b) Energy vs reaction coordination diagram, comparing kinetically constrained chemical processes between shock- and static-compressed materials, leading to decomposition and polymerization, respectively.
Dynamic-DAC
Gas-guns, high-power lasers, and magnetic drivers are also used in HP research to investigate material behaviors under dynamic compressions. The Z-pulse power machine and high-power lasers, such as the Omega (Ω) and the National Ignition Facility (NIF), extend the limit of static pressures to dynamic pressures, well over 1 TPa.
Shock and static high pressures are complimentary in many aspects, including in thermal conditions, kinetics, states of stress, and rates of loading; all of these can affect material transformations (
The complementary information from shock- and static-high-pressure experiments is critical to gain insight into material transformations at high PT conditions. Furthermore, combining these static and dynamic HP methods with advanced light sources, such as third-generation (3G) synchrotron x-rays, 4G x-ray free electron lasers (XFELs), and spallation neutron sources (SNS), provides opportunities to discover new materials and exploit new chemistry under extreme PT conditions.
3 III. STRUCTURE AND CHEMICAL BONDING
The nature of chemical bonding in a dense solid under extreme conditions can be quite different from that under ambient conditions, a nature manifested in the solid’s crystal structure. An excellent example of this is the nearly identical density between noble gas solid Xe and ionic solid CsI above ∼80 GPa–300 GPa,
To illustrate the pressure-induced evolution of structure and chemical bonding under extreme pressures, we consider a hypothetical 2D molecular lattice in
![A concept of pressure-induced chemistry in a 2D lattice, illustrating the evolution of chemical bonding and structure with increasing pressure, from weakly bound molecular solids to covalently bonded extended solids and ionic solids. These transformations are primarily driven by pressure-induced densification, promoting the delocalization and even ionization (or localization) of electrons from both valence and core states [Reproduced with permission from C.-S. Yoo, MRS Bull. 42, 724 (2017). Copyright 2017 Cambridge University Press].](/richHtml/mre/2020/5/1/018202/img_3.jpg)
Fig. 3. A concept of pressure-induced chemistry in a 2D lattice, illustrating the evolution of chemical bonding and structure with increasing pressure, from weakly bound molecular solids to covalently bonded extended solids and ionic solids. These transformations are primarily driven by pressure-induced densification, promoting the delocalization and even ionization (or localization) of electrons from both valence and core states [Reproduced with permission from C.-S. Yoo, MRS Bull. 42 , 724 (2017). Copyright 2017 Cambridge University Press].
Extended solids initially possess high-symmetry open structures, with highly degenerated atomic configurations, as found in diamond and symmetric ice X. With increasing compression, these open network structures are subjected to subtle structural symmetry-breaking distortions, which are electronic in origin. The driving force for symmetry lowering transitions is densification achieved by polarizing the chemical bonds. The consequence of structural distortion is, however, many near-ground states, providing pathways to local energy minima, path-dependent transitions, and phase metastability, as observed in layered polymeric nitrogen (LP-N)
Upon further compression, to well above 100 GPa, the valence electrons of low-Z extended solids may ionize to form an ionic solid or a mixture of substituent elemental solids. Pressure-induced ionization occurs as electrostatic forces begin to dominate at extreme conditions. At a given density, a delicate balance between electron delocalization (governing bonding) and ionization (governing packing) can give rise to novel structures and phenomena. Important properties resulting from this balance between bonding and packing include kinetic-controlled transformations, novel interfacial structures, and large bandgap ionic solids.
The HP chemistry described above occurs at large compression energies,
4 IV. PRESSURE-INDUCED ELECTRON DELOCALIZATION TO EXTENDED COVALENT SOLIDS
Pressure-induced transformations from dense molecular solids to nonmolecular extended solids with strong covalent-bond frameworks reveal a fundamental principle of high-pressure chemistry; that is, pressure-induced electron delocalization, which occurs because electron kinetic energy has a higher density dependence (ρ2/3) than does electrostatic potential energy (ρ1/3). As a result, electrons localized within intramolecular bonds become increasingly less stable as density (or pressure) increases, and the intermolecular potential becomes highly repulsive. At high enough pressures, this leads to more electron-delocalized states, such as polymeric or metallic solid states. This is the reason that many unsaturated molecular bonds become unstable at pressures of 10–20 GPa,
The pressure-induced electron delocalization in dense molecular solids can be understood in terms of storing large compression energies into chemical energies in dense framework structures. The resulting materials typically have high densities (3–4 g/cm3) and high energy densities (1–10 eV/nm), constituting a high-energy-density solid (HEDS). Because a HEDS is made of strong covalent bonds, there can be a large kinetic barrier to depolymerization, providing opportunities to recover them at ambient conditions. This is illustrated in
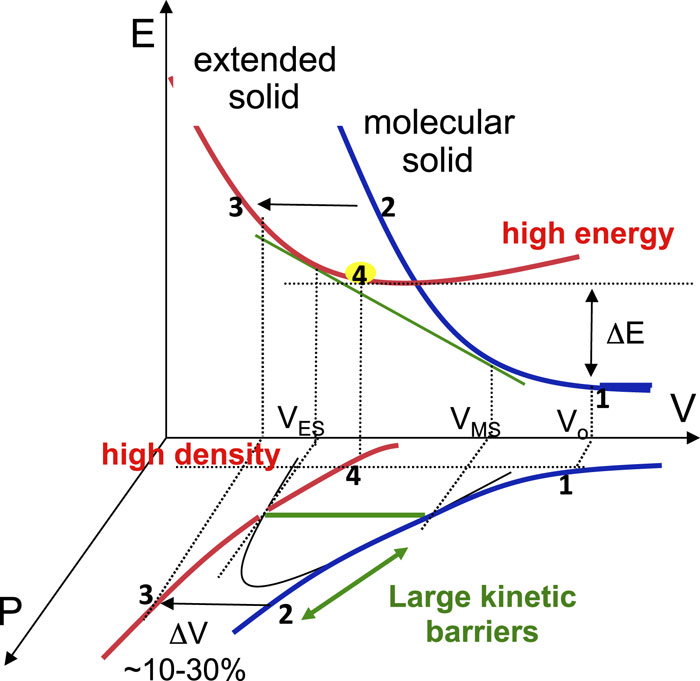
Fig. 4. A thermodynamic representation of pressure-induced electron delocalization to high density, high energy extended solids. Vo signifies the specific volume of molecular solid under ambient conditions, whereas VES and VMS represent the molecular solid and extended solid at the transition pressure. The pressure (or energy) offset of the transition (2 → 3) from the equilibrium value (the green line) signifies the presence of a large activation barrier. A similar kinetic barrier in the backward transition (3 → 4), on the other hand, makes it possible to recover the high-energy, high-density product under ambient conditions.
Nitrogen was the first element predicted to form singly bonded cg-N,
Low-Z extended solids exhibit many interesting mechanical, optical, and electronic properties, in addition to high energy density, and constitute a class of novel materials. Some examples are shown in
![Novel properties observed in low-Z extended solids: (a) Second harmonic generation of silica-like CO2-V. (b) Superconductivity of dense CS2. (c) High energy density of polymeric CO. (d) Colossal Raman scattering of layered polymeric nitrogen (LP-N) [Reproduced with permission from C.-S. Yoo, MRS Bull. 42, 724 (2017). Copyright 2017 Materials Research Society].](/richHtml/mre/2020/5/1/018202/img_5.jpg)
Fig. 5. Novel properties observed in low-Z extended solids: (a) Second harmonic generation of silica-like CO2-V. (b) Superconductivity of dense CS2. (c) High energy density of polymeric CO. (d) Colossal Raman scattering of layered polymeric nitrogen (LP-N) [Reproduced with permission from C.-S. Yoo, MRS Bull. 42 , 724 (2017). Copyright 2017 Materials Research Society].
5 V. PRESSURE-INDUCED ELECTRON LOCALIZATION TO LOW SYMMETRY IONIC SOLIDS
The formation of nonmolecular extended solids produces relatively highly symmetric local structures of corner-shared polyhedra. With regard to density, these are relatively open structures that are eventually subjected to lattice distortions in tighter spaces or under further compression, to pressures well above 100 GPa. Driven by densification, the valence electrons of extended solids may become highly polarized and even ionized to form ionic solids, as the columbic attraction supersedes the stabilization of electron delocalization (bonding) at small interatomic distances. As a result, the high-symmetry extended solids in the 3D covalent networks transform into ionic solids with highly distorted, low-symmetry and low-dimension structures, as predicted and observed in H2O, N2, CO, and CO2 at high pressure.
Evidence for pressure-induced ionization can be found in the layered structures of dense solids at extreme pressures (
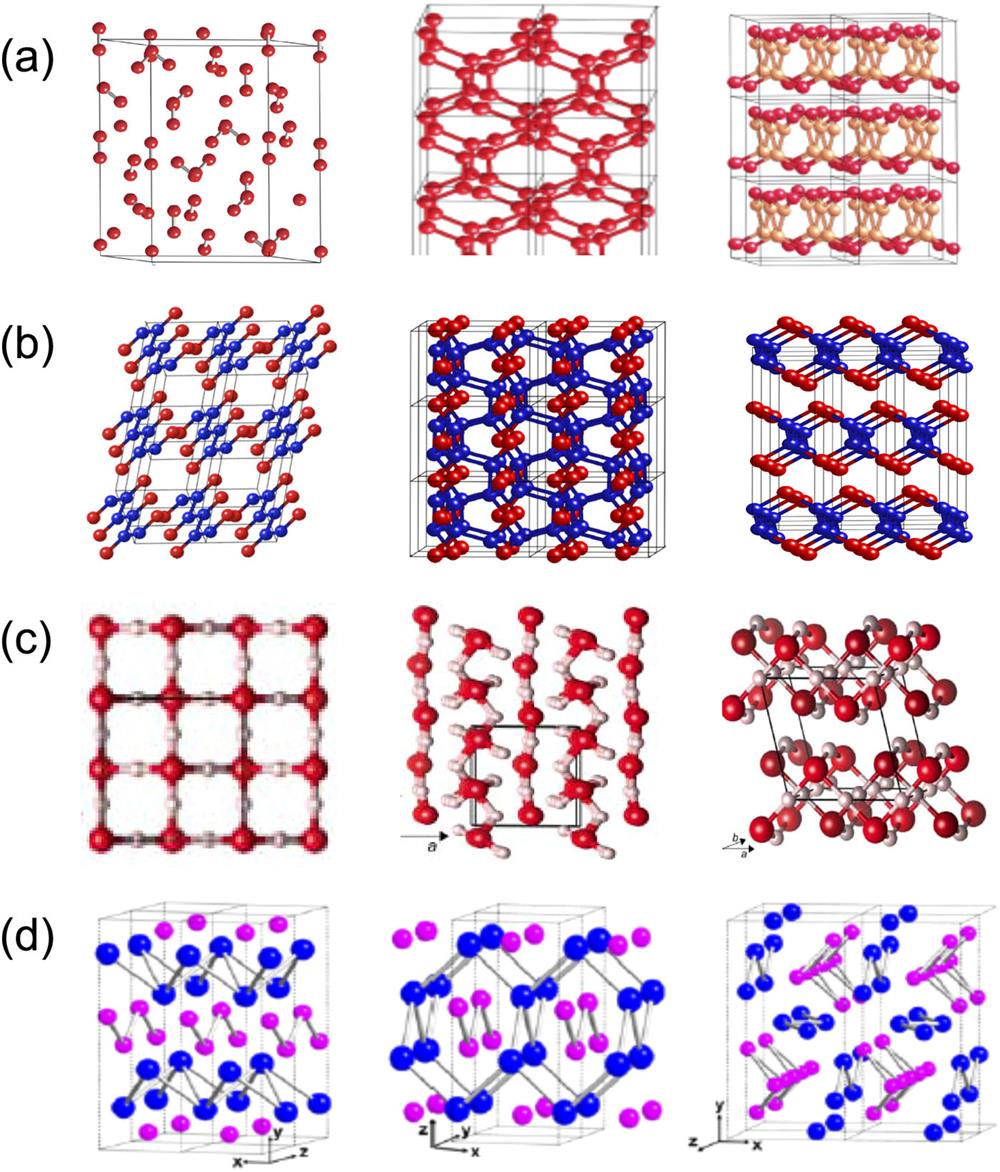
Fig. 6. Crystal structures of dense low-Z solids under extreme conditions, showing the pressure-induced ionization of 3D covalent bonds to 2D ionic solids in: (a) N2 from the molecular ε(r- 3m ) phase to the singly bonded phase, 3D cg -N (I 213) at 110 GPa, and 2D layered polymeric LP-N (Pba 2) at 150 GPa. (b) CO from 1D linear chain phase I (P 21/m ) to 3D ladder phase II (P 212121) and 2D layered phase III (Cmcm ). (c) H2O from 3D symmetric ice X (Pn- 3m ) at 80 GPa to distorted P 21 phase at 1 TPa and 2D layered C 2/m at 2 TPa. (d) NaCl from an oC 8 phase at 320 GPa to oI 8 at 650 GPa and oP14 above 700 GPa.
The formation of similar, layered structures has also been observed in dense CO, but at substantially lower pressures of 50–100 GPa.
The evidence of pressure-induced ionization is found not only in molecular solids, CO and N2, with unsaturated bonds, but also in singly bonded H2O [
The low-pressure B1 (Fm3m or cF8) phase of NaCl transforms into a CsCl-like B2 (Pm3m or cP2) phase at ∼30 GPa,
Clearly, there is abundant evidence for pressure-induced ionization to form low-symmetry, yet high-density, layered structures in dense solids, regardless of the nature of the chemical bonding that includes those existing as molecular solids, ionic solids, and even noble-gas solids at low pressures. Therefore, it is conceivable that the symmetry lowering transition from a 3D framework structure to a 2D layered structure occurs more often, in general, following the formation of extended covalent network solids. It arises from a substantially enhanced electrostatic (i.e., coulombic) interaction in densely packed solids at extreme pressures, well above 100–300 GPa. In fact, the formation of simple metal electrides
6 VI. METALLIZATION OF IONIC SOLIDS
One important question is whether an ionic state, such as pressure-induced ionization, could ever be metallized? If so, when (pressure), how (mechanism), and where (states) would it transform? This is a crucial but unresolved question in relation to most materials because of experimental limitations in achieving those extreme conditions that are only within the realm of theory. Nevertheless, the metallization of ionic solid CsI in comparison with the van der Waals noble-gas solid Xe may offer some insights into the chemical mechanism of metallization in dense ionic solids. For example, the B2 (Pm3m) phase of CsI transforms into an orthorhombic (Pnma) phase at 42 GPa, with a hexagonal, closed packed (hcp) stacking,
It is important to note that, despite the closed-shell structure, valence electrons in Xe are highly polarizable, which can give rise to a wide range of chemical compounds.
In NaCl [
Novel metallic states are also expected to form from dense ionic phases of N2 and H2O (P41), but at even higher pressures. The metallic state of solid N2 has not yet been predicted, although increasing optical reflectivity has recently been reported in fluids above 2500 K and 125 GPa
7 VII. TEMPERATURE-INDUCED IONIZATION
Increasing temperature expands the bond distance in more open structures like bcc because of a large increase in entropy

Fig. 7. A conceptual phase/chemical transformation diagram of molecular solids, signifying the pressure and temperature induced ionization leading to nonmolecular polymeric, metallic, and ionic solids. A delicate balance between the compression energy (PΔV) and the entropic energy (TΔS) under extreme pressure-temperature conditions gives rise to novel states and transitions of materials in both solids and liquids, which are strongly controlled by chemical kinetics. The compression energy at 100 GPa (∼10 eV) can strongly modify the valence electron configuration or chemical bonds of molecular solids; at 1 TPa (∼100 eV) it can even alter the core electron configuration and induce a new type of chemistry never experienced in the past.
At high temperatures, materials can increase their fluidity (or diffusivity) by a substantial degree, especially if they are light elements, such as H and He, and form novel superionic states of dense solid H2O and NH3, where hydrogen atoms can move freely, while oxygen atoms are fixed in their anionic sublattice. Recently, it has been predicted that He and H2O can also form stable compounds, such as He(H2O)x where x = 1, 2, 3,… over a large pressure range—even close to ambient pressure.
8 VIII. KINETIC-CONTROLLED TRANSFORMATIONS
Kinetically controlled processes provide opportunities to observe metastable phases and develop new materials, both crystalline and amorphous solids, under rapid compression using dynamic-DAC and rapid quenching using laser-heated DAC, as well as by shock compression using gas guns and high-power lasers. This approach stems from the fact that the dynamic shear strains generated by rapid yet precisely controlled pressure (or temperature) modulations can enhance interfacial mixing and thereby the chemistry between the dislike lattices of C, B, N2, H2, and O2. Furthermore, by precisely controlling pressure, large crystals can be grown along the melt boundary into different crystal morphologies, including single crystals, fractals, and dendrites, by varying the characteristics of pressure modulation, and the shape, frequency, amplitude, and compression rate. This is observed during the solidification of water, as shown in
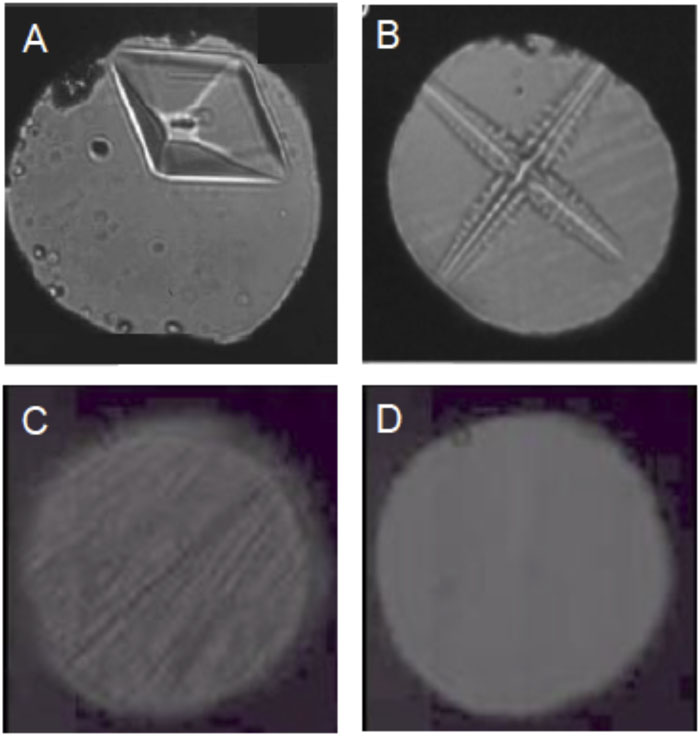
Fig. 8. Various ice crystals, stable and metastable, formed along different thermal and kinetic path under rapidly modulating pressures using dynamic -DAC, including (a) single crystal ice VI (b) dendritic ice VI (c) metastable ice VII produced in the stability field of ice VI, and (d) high density amorphous (HDA) ice produced in no man’s land.
The transition mechanisms of dense solids are generally complex and often controlled by strong kinetics, especially in the transition regions of: (i) Structural miscibility at relatively low pressures,
9 IX. DENSE FRAMEWORK SOLIDS WITH HIGH CHEMICAL ENERGIES
Discoveries of novel low-Z extended solids at high pressures have opened up new avenues of research inquiry. While these covalently bonded extended framework solids exhibit unusual properties, such as high energy density, extreme hardness, second harmonic generation, a colossal Raman cross section, and a record high Tc superconductivity (
The development of low-Z extended solids amenable to ambient stabilization poses great scientific and technological challenges. Those challenges primarily stem from the formidable transition pressures and high-energy states, which make them metastable at low pressures. A logical way to overcome these challenges is to use low-Z solid mixtures, where the internal chemical pressure can lower the transition pressure, and strong hetero-nuclear chemical bonding replaces dangling bonds, enhancing the stability of extended network structures under ambient conditions. Furthermore, a greater number of new materials can potentially be developed via solid-state combinatory reactions of mixtures. Importantly, the use of mixtures permits the control of the transition pathway and pressure, and of the stability, structure, and properties of products.
The novel properties of extended solids (
![Photographic images of polymeric carbon monoxide (pCO) products under ambient conditions, recovered after synthesis at high pressures: (a)–(c) pure pCO synthesized at 8, 9, and 10 GPa. The images show the presence of two polymeric products (I and II) (d) and (e) 10% H2 doped pCO synthesized at 6 and 7 GPa, showing lower synthetic pressures than pure CO and strong luminescence [Reprinted with permission from Y. J. Ryu et al., J. Phys. Chem. C 121, 10078 (2017). Copyright (2017) American Chemical Society].](/richHtml/mre/2020/5/1/018202/img_9.jpg)
Fig. 9. Photographic images of polymeric carbon monoxide (pCO) products under ambient conditions, recovered after synthesis at high pressures: (a)–(c) pure pCO synthesized at 8, 9, and 10 GPa. The images show the presence of two polymeric products (I and II) (d) and (e) 10% H2 doped pCO synthesized at 6 and 7 GPa, showing lower synthetic pressures than pure CO and strong luminescence [Reprinted with permission from Y. J. Ryu et al. , J. Phys. Chem. C 121 , 10078 (2017). Copyright (2017) American Chemical Society].
![Pressure-volume compression curves of extended (red circles) and molecular (green triangles and yellow squares) CON2 phases in comparison with those of cg-N (blue line), LP-N (brown), δ-N2 (yellow), and ε-N2 (green). The inset shows the crystal structure of P43 solid, consisting of four-fold coordinated carbon atoms (brown), three-fold nitrogen (gray), and two-fold oxygen (red) in a three-dimensional framework structure [Reprinted with permission from C.-S. Yoo et al., J. Phys. Chem. C 122, 13054 (2018). Copyright (2017) American Chemical Society].](/richHtml/mre/2020/5/1/018202/img_10.jpg)
Fig. 10. Pressure-volume compression curves of extended (red circles) and molecular (green triangles and yellow squares) CON2 phases in comparison with those of cg -N (blue line), LP-N (brown), δ-N2 (yellow), and ε-N2 (green). The inset shows the crystal structure of P 43 solid, consisting of four-fold coordinated carbon atoms (brown), three-fold nitrogen (gray), and two-fold oxygen (red) in a three-dimensional framework structure [Reprinted with permission from C.-S. Yoo et al. , J. Phys. Chem. C 122 , 13054 (2018). Copyright (2017) American Chemical Society].
The formation of CON2 copolymer strongly suggests that a concerted reaction mechanism is in play [
![Phase diagram of Fe(CO)5, consisting of liquid, three molecular phases (noted as I, II, and III), and a polymeric product. Note that unreacted Fe(CO)5 is stable within a limited pressure-temperature range, reflecting the weakness of Fe–CO back bonds with increasing temperatures. The inset shows the lustrous appearance of recovered Fe(CO)5 polymer, coming from a graphite-like 2D carbon-oxygen polymer on the surface [Reprinted with permission from Y. J. Ryu et al., Sci. Rep. 5, 15139 (2015). Copyright 2015 Macmillan Publishers Ltd.].](/richHtml/mre/2020/5/1/018202/img_11.jpg)
Fig. 11. Phase diagram of Fe(CO)5, consisting of liquid, three molecular phases (noted as I, II, and III), and a polymeric product. Note that unreacted Fe(CO)5 is stable within a limited pressure-temperature range, reflecting the weakness of Fe–CO back bonds with increasing temperatures. The inset shows the lustrous appearance of recovered Fe(CO)5 polymer, coming from a graphite-like 2D carbon-oxygen polymer on the surface [Reprinted with permission from Y. J. Ryu et al. , Sci. Rep. 5 , 15139 (2015). Copyright 2015 Macmillan Publishers Ltd.].
Interest in 3d metal-doped low-Z extended solids centers on the goal of stabilizing highly metastable low-Z network structures by forming metal low-Z organic bonds, while harvesting the functional properties of 3d transition metals and unusual mechanical properties of dense metal-organic framework (deMOF) materials. The variety in electron polarity, structure, strength, and bond energy of metal-organic bonds, as well as the catalytic effect of metal species, can provide alternative reaction pathways to low-Z extended solids at more practically viable pressures and give rise to novel functional properties from doped metals, such as electric conductivity or magnetism.
10 X. CONCLUDING REMARKS
The recent discoveries of novel states and new materials under extreme conditions reveal several fundamental concepts governing the chemistry of dense solids under extreme conditions. These include the pressure evolution of chemical bonding and structure in dense solids from molecular solids to covalent, ionic, and, ultimately, metallic solids, as well as the novel properties and complex transition mechanisms arising from the subtle balance between the electron hybridization (bonding) and electrostatic interaction (packing) in densely packed solids. We have emphasized that, while many of these transitions are predictive based on thermodynamic constraints, the mechanisms are rather complex and are often controlled by strong kinetics. Especially important are the kinetic controlled processes in the transition regions of structural miscibility at relatively low pressures, chemical bonding in an intermediate-pressure range, and electrostatic packing at extremely high pressures. A profound knowledge gap in both theory and experiment motivates future studies on the novel states, transformations, and chemical mechanisms of dense solids in these transition regions.
Modern advances in experimental and computational capabilities bring us closer to the goal of developing new materials on the basis of computational design coupled with experimental discovery and characterization. The application of large compression energies, comparable to chemical bond energies in solids, can advance the concept of materials by design in unique ways by converting molecular solids into novel 3D network structures that can be predicted on the basis of first principles and materials data-based computational science. This control and manipulation of chemical bonding is particularly effective in the case of solids made from low-Z elements, in which thermo-mechanical energies rapidly become substantially larger than those associated with the formation and diffusion of defects and grain boundaries. Importantly, these dense framework solids, when made of low-Z elements, are intrinsically hard yet light, e.g., diamond and c-BN, and exhibit superior thermal, mechanical, chemical, and electro-optical properties. Thus, they constitute the next generation of structural materials that are dense, light, multifunctional frameworks.
With modern advances in high-pressure and laser technologies, it is now possible to achieve the extreme PT conditions of the giant planets and the large compressions that force materials into fundamentally new physical and chemical configurations. Thus, the chemistry concepts presented in this paper have important implications with regard to the development of new planetary models, condensed materials theories, and extreme chemistries involving deep core electrons.
[1] . Structure of exoplanets. Proc. Natl. Acad. Sci. U. S. A., 2014, 111: 12622-12627.
[2] . Global inventory of methane clathrate. Earth Planet. Sci. Lett., 2004, 227: 185-199.
[6] . Man-made diamonds. Nature, 1955, 176: 51-55.
[7] . Polymeric nitrogen. Phys. Rev. B, 1992, 46: 14419-14435.
[8] . Single bonded cubic form of nitrogen. Nat. Mater., 2004, 3: 558-563.
[13] . Structure of polymeric carbon dioxide CO2-V. Phys. Rev. Lett., 2012, 108: 125701-1-125701-4.
[14] . Pressure effects on the hydrogen bond in ice up to 80. GPa, 1994, 322: 279-286.
[17] . Metallic hydrogen: A high-temperature superconductor?. Phys. Rev. Lett., 1968, 21: 1748-1749.
[18] . Conductive dense hydrogen. Nat. Mater., 2011, 10: 927-931.
[22] . Aluminium at terapascal pressures. Nat. Mater., 2010, 9: 624-627.
[26] . The revealing role of pressure in the condensed matter. Phys. Today, 1998, 51(8): 26-32.
[28] . High-pressure phases of silane. Phys. Rev. Lett., 2006, 97: 045504--4.
[29] . Materials discovery at high pressures. Nat. Rev. Mater., 2017, 2: 17005.
[38] . Moissanite: A window for high-pressure experiements. Science, 2000, 290: 783-785.
[41] . Novel development of high-pressure synthetic diamonds “ultra-hard nano-polycrystalline diamonds”. SEI Technol. Rev., 2012, 74: 15-22.
[51] . Ramp compression of diamond to five terapascals. Nature, 2014, 511: 330-333.
[52] . Insulator-metal transition in dense fluid deuterium. Science, 2018, 361: 677-682.
[53] . Probing matter at Gbar pressures at the NIF. High Energy Density Phys., 2014, 10: 27-34.
[68] . Equation of state and metallization of CsI. Phys. Rev. B, 1984, 29: 2611-2621.
[73] . Metallic CsI at pressures of up to 220 gigapascals. Science, 1998, 281: 1333-1335.
[76] . Graphite at pressure up to 55 GPa: Optical properties and Raman scattering-amorphous carbon?. Sov. Phys. JETP, 1989, 69: 380-381.
[82]
[84] . Bonding changes in compressed superhard graphite. Science, 2003, 302: 425-427.
[85] . Synthesis of quenchable amorphous diamond. Nat. Commun., 2017, 18: 322-1-322-7.
[88] . Singe-crystal x-ray diffraction study of β nitrogen. J. Chem. Phys., 1962, 37: 2962-2965.
[93] . High-energy-density extended CO solid. Nat. Mater., 2005, 4: 211-215.
[95] . Prediction of new low compressibility solids. Science, 1989, 245: 841-842.
[96] . Transition temperature of strong-coupled superconductors. Phys. Rev., 1968, 167: 331-344.
[97] . The structure of N2 at 49 kbar and 299 K. Acta Crystallogr. B, 1981, 37: 8-11.
[100] . High-pressure amorphous nitrogen. Phys. Rev. B, 2001, 64: 052103-1-052103-4.
[101] . High-pressure phases of nitrogen. Phys. Rev. Lett., 2009, 102: 125702-1-125702-4.
[115]
[116] . High pressure ices. Proc. Natl. Acad. Sci. U. S. A., 2012, 109: 745-750.
[117] . High pressure partially ionic phase of water ice. Nat. Commun., 2011, 2: 563-1-563-5.
[118] . New high-pressure phases of ice. Phys. Rev. Lett., 1988, 60: 2284-2287.
[119] . Pressure-induced phase transformation in NaCl. J. Appl. Phys., 1968, 39: 319-325.
[128] . New high-pressure phases of lithium. Nature, 2000, 408: 174-178.
[129] , et al.. Transparent dense sodium. Nature, 2009, 458: 182-185e.
[132]
[138] . Multiple superionic states in helium-water compounds. Nat. Phys., 2019, 15: 1065.
[147]
[148] . Coesite-like CO2: An analog to SiO2. Phys. Rev. B, 2010, 82: 012105-1-012105-4.
[154] . Azole-based energetic salts. Chem. Rev., 2011, 111: 7377-7436.
[156] . Exploring high-pressure structure of N2CO. J. Phys. Chem. C, 2014, 118: 27252-27257.
[163] . Porous, crystalline, covalent organic frameworks. Science, 2005, 310: 1166-1170.
Article Outline
Choong-Shik Yoo. Chemistry under extreme conditions: Pressure evolution of chemical bonding and structure in dense solids[J]. Matter and Radiation at Extremes, 2020, 5(1): 018202.