Enhancing plasmonic trapping with a perfect radially polarized beam
Download: 676次
1. INTRODUCTION
Optical traps, originating from forces induced by electromagnetic fields, have been extensively used as a powerful scientific tool to study the physical, chemical, and biological characteristics of micro-/nano-objects [1,2]. Metal particles, based on their special chemical and physical properties, possess extensive applications in various areas including spectroscopy, catalysis, and bio-/medical science and techniques [3]. However, it is well known that metallic particles are hard to trap by traditional optical tweezers due to their high absorption and reflection features. Recently, many approaches have been proposed to enhance the gradient force or suppress the scattering force to increase the trapping efficiency, such as trapping of Rayleigh particles [4,5] or employing plasmonic nanostructures [68" target="_self" style="display: inline;">–
To achieve high performance of the plasmonic tweezers in such applications, the trapping stiffness is a key issue. Stable trapping and manipulation of particles are in high demand in nanofabrication, sensing, and quantum technologies, which actively promotes the development of techniques for trapping stiffness improvement. However, plasmonic trapping stiffness is inevitably affected in various terms from the Brownian movement to thermal convection [18]. The most direct way to enhance the stiffness is by reducing the negative heating effect; thus, cooling has become a popular method and has been widely used in trapping systems [19,20]. Nevertheless, the cooling module is always cumbersome, and in the all-optically excited SPP trapping systems, the thermal effect is not strong [9], resulting in a limited improvement in trapping stiffness. Besides cooling, another candidate for stiffness enhancement is by enhancing the trapping gradient force and suppressing the repulsive scattering force, which requires refined designs of the excited plasmonic fields as well as the incident light beams.
In this work, we investigate how to reduce the scattering force and enhance the plasmonic trapping stiffness through a novel perfect radially polarized beam (PRPB) [21]. Although the radially polarized beam (RPB) is an effective way to excite the focused SPP field for trapping [9], its directly transmitted light through the thin metallic film causes a strong scattering force acting on the particle to strengthen the Brownian movement. Here, based on theoretical and experimental studies, we demonstrate an effective method for improving plasmonic trapping stiffness by employing two axicons to compress the incident RPB into a PRPB. The whole energy of the generated PRPB is confined into a very sharp ring with tunable diameter to fit the SPP exciting angle. Consequently, most direct transmission through the metallic film is suppressed, while the exciting efficiency of SPP is increased to provide a stronger trapping force. The experimental results verify the enhancement of the trapping stiffness and present the reduced Brownian movement. We believe that this method could become a very practical way for enhancing the performance of plasmonic trapping and manipulation in chemical and physical lab-on-a-chip research.
2. EXPERIMENTAL SETUP AND METHODS
In the all-optically excited SPP tweezers [4], RPB is usually employed due to its high SPP coupling efficiency compared with other polarizations [22]. However, when the RPB is tightly focused onto the metal film by a high-numerical-aperture (NA) objective, the SPP is only excited at the strict angle that satisfies the wavevector matching condition. The light of the RPB at other angles is reflected, absorbed, or transmitted by the metal film, where the transmitted part would increase the scattering force and the absorbed part would generate heat, both decreasing the trapping stiffness. To reduce the influences of the transmitted and absorbed lights, there is a simple choice to block the inner transmitted part of the RPB [23]; however, most of the energy is wasted and the SPP exciting efficiency is not improved. To further enhance the SPP exciting efficiency, the best choice is to concentrate the whole energy of the light into a very sharp ring to fit the SPP exciting angle.
The recently developed perfect optical vortices (POVs) provide a good candidate that can confine all incident energy into a sharp bright ring with a tunable radius independent on the topological charge [2426" target="_self" style="display: inline;">–
Herein, a PRPB-based plasmonic tweezers configuration is proposed that employs two axicons to compress the incident RPB into a thin PRPB for establishing a stiffness enhanced trap, as shown in Fig.
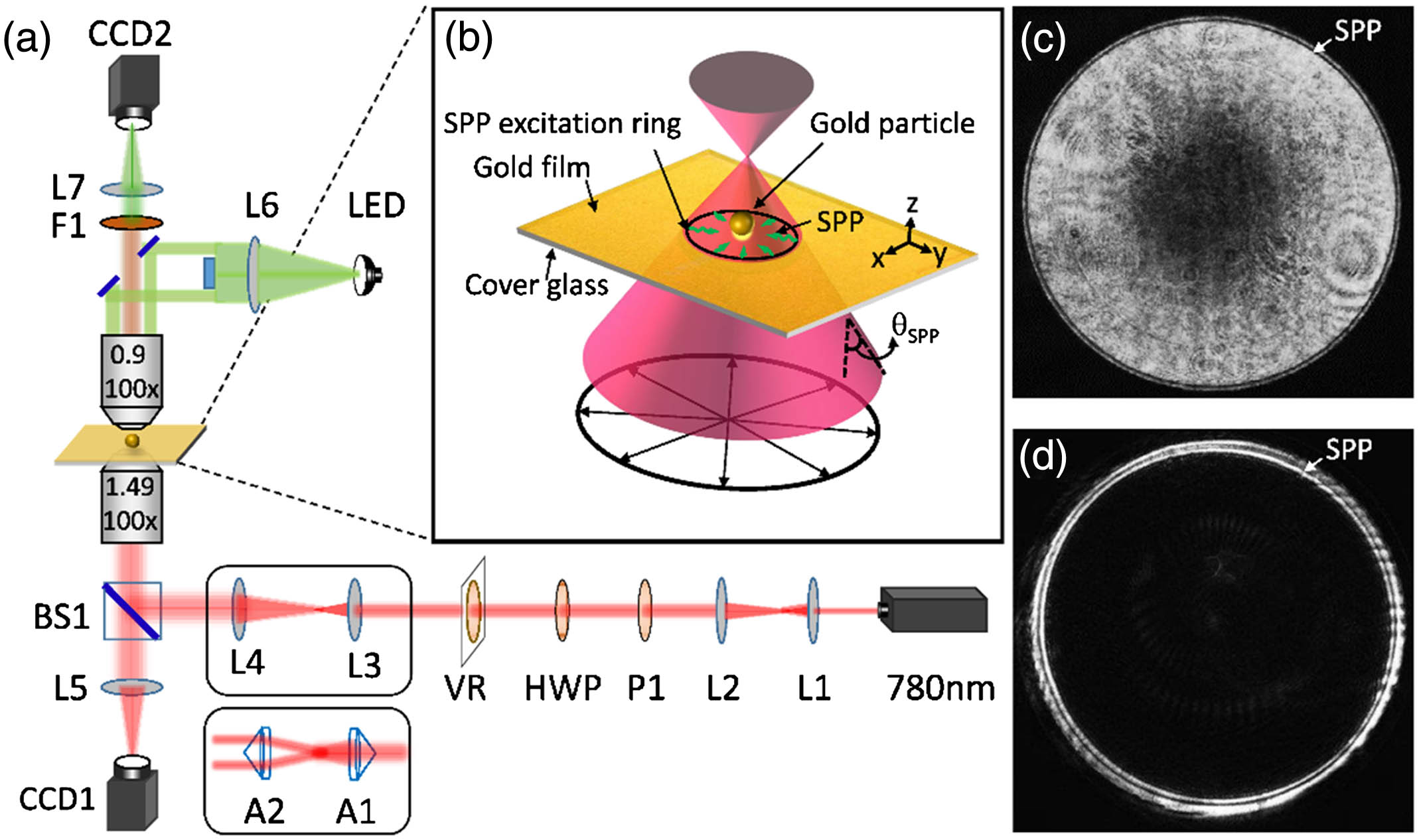
Fig. 1. Dynamic plasmonic tweezer system construction and two types of excitation optical beam generation. (a) Technical schematic of the generating RPB and PRPB for the optical tweezer system. The RPB generated by vortex retarder (VR) is also the PRPB generated by changing telescope system (L3, L4) with two axicons (A1, A2). (b) Technical schematic of the SPP excitation process for a focusing RPB. The black arrows indicate the radial polarization directions. (c) The profile of the reflected light obtained at the back focal plane for RPB. (e) The profile of the reflected light obtained at the back focal plane for PRPB.
The generated RPB or PRPB is then tightly focused by an objective (
Figure
The profiles of reflected light at the back focal plane can be obtained by CCD1 in Fig.
3. RESULTS AND DISCUSSION
To demonstrate that the PRPB is more efficient than the RPB in a plasmonic trap, the finite difference time-domain (FDTD) method is used to calculate the electric field distributions of the SPP at the gold–water interface. As shown in Figs.

Fig. 2. Calculated electric field intensities at the gold–water interface for focused RPB and PRPB. (a), (b), (c) Cross-section distribution and focused state for RPB, PRPB and RPB with no SPP excitation mode. (d), (g) Electric field intensities at the gold–water interface (horizontal plane) and in the plane for RPB. (e), (h) Electric field intensities at the gold–water interface ( plane) and in the plane for the PRPB. (f), (i) Electric field intensities at the gold–water interface ( plane) and in the plane for the RPB with no SPP excitation mode. The white lines in the bottom of (g), (h), and (i) indicate the gold–water interface.
Figures
Additionally, the optical forces acting on the particle located in both fields are calculated through the Maxwell stress tensor method [30]. All structures and parameters chosen in the calculation are exactly according to the experimental conditions, i.e., the gold film thickness is set to be 50 nm, the diameter of the particle is 1 μm, and the gap between the particle and the gold film is 10 nm. Figure

Fig. 3. Calculated force distributions at the gold–water interface for the focused RPB and PRPB. (a) Distributing curve of force for gold particles in the radial direction with the RPB and PRPB. (b) Distributing curve of force for gold particles in axial direction with the RPB and PRPB.
Trapping stiffness is an appropriate quantity to illustrate the efficiency of optical tweezers [2]. To verify the numerical studies above, trapping experiments were implemented, and the dynamic processes were recorded for trapping stiffness analysis. Traditionally, a quadrant photodiode (QPD) was usually used to measure the position of trapped particles and analyze the trapping stiffness [2,31], as it can offer precise and high-bandwidth measurements with typical frequencies of several kilohertz (kHz). However, a QPD is not suitable for the plasmonic tweezers here. Due to the high absorption and reflection of incident light by the gold film, only a small part of light can transmit through the film, especially for the PRPB. Therefore, there is not enough scattering light captured by QPD, which could affect the sensitivity of particle localization. Consequently, in the experiment we chose another approach based on a high-speed video camera to measure the particle position and force, which is also widely used [31–
To retrieve the motion data from the image sequences, an image registration technique, phase correlation (PC) [35] of the Fourier transform method, is adopted to achieve sub-pixel motion resolution [33]. Figures
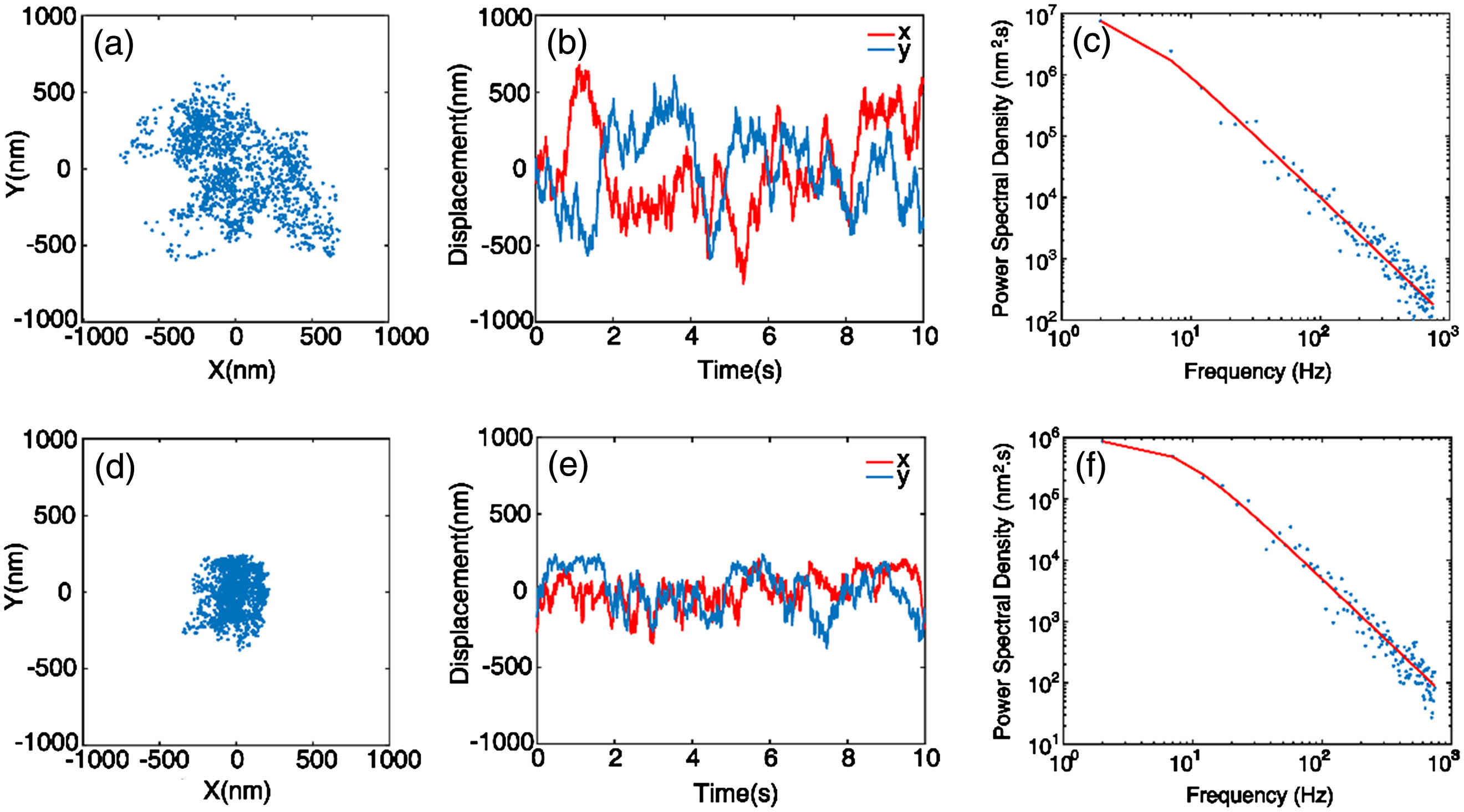
Fig. 4. Position tracking and power spectra analysis of the trapped gold particles with a diameter of for (a)–(c) RPB and (d)–(f) PRPB. The laser powers at the BFP are about 13.9 mW and 12.4 mW for the RPB and PRPB, respectively. (a) Scattering distribution of the position for the gold particle in RPB. (b) Displacement of the particle versus time for RPB. (c) The power spectra of the particle for RPB fitting with a Lorentzian curve. (d) Scattering distribution of the position for the gold particle in PRPB. (e) Displacement of the particle versus time for PRPB. (f) The power spectra of the particle for PRPB fitting with a Lorentzian curve.
It is well known that a trapping force exerted on particles can be considered following Hooke’s law, where trap force is proportional to the displacement with a stiffness constant
Finally, to further demonstrate the trapping ability of the PRPB, we measured the transverse trapping stiffness of the RPB and PRPB under different incident laser powers and particle diameters, as shown in Figs.

Fig. 5. Trapping stiffness as a function of laser power and particle diameter. (a) Transverse trapping stiffness as a function of laser power for 1 μm gold particles trapped by RPB and PRPB. (b) Transverse trapping stiffness as a function of gold particles diameter for laser power at 12.4 mW trapped by RPB and PRPB.
Both theoretical and experimental results demonstrate that a PRPB with a tunable sharp ring can reduce the scattering force on the trapped particle and enhance the plasmonic field as well as trapping stiffness. Consequently, a PRPB is more efficient than an RPB in exciting SPP tweezers.
4. CONCLUSIONS
In conclusion, through adding an axicon pair in a conventional dynamic SPP optical tweezer system with RPB, we successfully constructed a more efficienct plasmonic tweezers system with PRPB. The ring-shaped PRPB can be tuned such that the power of the beam is mainly concentrated at the SPP exciting angle under a tight focusing configuration, while the direct transmission light through the metallic film was suppressed. We have theoretically and experimentally demonstrated that the PRPB has a higher efficiency in trapping metal particles than RPB, and significantly improves the excitation efficiency of SPP. PRPB has shown high efficiency both on particle trapping and field enhancement, which provides great potential in many applications, such as dynamic SERS measurement and imaging, nanostructure fabrication, and lab-on-a-chip research.
Article Outline
Xianyou Wang, Yuquan Zhang, Yanmeng Dai, Changjun Min, Xiaocong Yuan. Enhancing plasmonic trapping with a perfect radially polarized beam[J]. Photonics Research, 2018, 6(9): 09000847.