Semiconductor lasers for high-speed information technologies (Invited Paper)
Download: 1092次
The concept of a laser was first proposed by Shawlow and Townes in 1958[1]. A few years later, Hall
In this Review, the development of semiconductor lasers for optical communications and information technologies is reviewed. A possible category of semiconductor lasers is introduced based on their different applications in optical communication systems. Different types of semiconductor lasers are discussed in terms of working principle, development history, advantages, and limitations.
Figure
The basis of the semiconductor laser is lasing of light. The first semiconductor laser operated in pulse due to the homo-junction structures grown on GaAs substrates[5]. In 1970, the use of hetero-junctions led to the realization of the first continuous wave (CW) semiconductor laser, which was reported by Alferov
Figure
Optical fiber communication was proposed in the mid-1960s by Kao and Hockham[8]. Multi-mode lasers, such as Fabry–Perot (FP) cavity lasers[9], can be used for data transmission in a multi-mode fiber. However, they suffer from high dispersion in an optical fiber, which makes it unsuitable for long haul transmission. To overcome the limitations induced by a poor and wide spectrum of FP lasers, the single mode laser is proposed. The introduction of Bragg grating increases the side mode suppression ratio and generates single longitudinal mode emission, which is the so-called distributed feedback (DFB) laser[10]. In 1973, Nakamura
The development of semiconductor lasers mainly spreads over three directions, i.e. high frequency response, high spectral quality, and high output power, which is illustrated in Fig.

Fig. 4. 3D coordinate shows the relationship of different types of semiconductor lasers for different applications.
For optical transmissions, a commercial DML has to be well packed to achieve a stable operation. In 1994, Morton
![(a) Our DFB module and (b) its frequency response at a different driving current[27].](/richHtml/col/2017/15/1/010002/img_005.jpg)
Fig. 5. (a) Our DFB module and (b) its frequency response at a different driving current[27].
The first vertical cavity surface emitting laser (VCSEL) was proposed by Iga in 1977[28]. Soon after, the first device was fabricated in 1979. Figure
For a high-speed DML, the
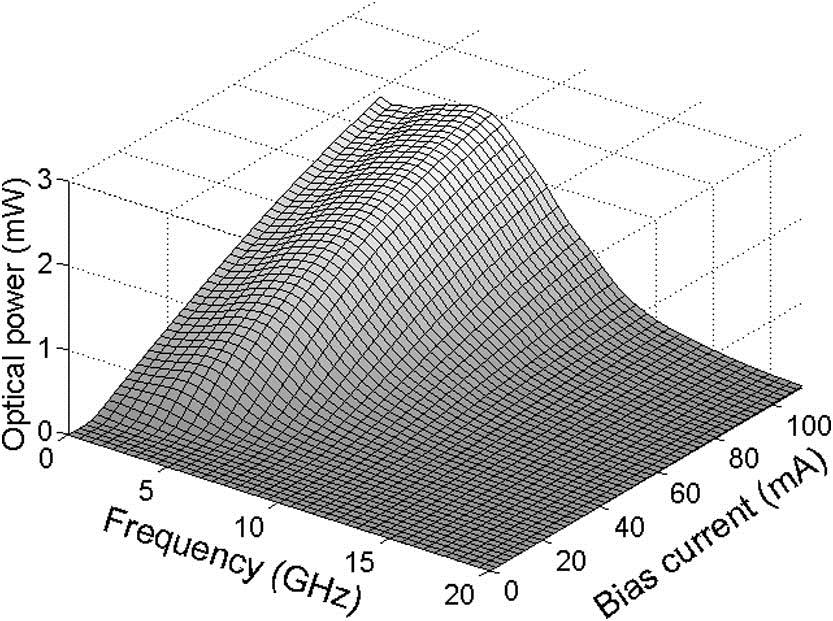
Fig. 7. Dynamic P-I curves for semiconductor lasers at a different modulation frequency.
High-speed modulation of an optical signal can also be realized using the external modulation of an optical carrier using external modulators. Single mode lasers can monolithically integrate with an electro-absorption modulator (EAM) or a Mach–Zehnder modulator (MZM) to realize the simplest photonic integrated circuit (PIC). The EAM modulates an optical carrier by absorbing the signal from the laser, which is realized by changing the absorption edge of the EAM with a driving signal. In 1986, the monolithic integration of a DFB laser and an EAM was demonstrated by Kawamura[32]. The modulation bandwidth is 2.5 GHz. A DFB laser integrated with an EAM was reported in 1997[33] and 2008[34].
In 1996, the first integration of a DFB laser and an interferometric MZM was reported by Adams
The second category of semiconductor lasers is related to the WDM system. The modulation speed of light increases four times in five years in synchronous digital hierarchy (SDH) and around 10 times in three years in the Ethernet. The bandwidth of the semiconductor laser suffers from two factors, relaxation oscillation frequency and parasitic impedance, which are mainly determined by the laser material. Since the modulation speed is hard to be improved, the WDM system is proposed to overcome this bottleneck. Different wavelengths of optical signals transmit in a single fiber, where significant improvement of transmission capacity is realized. Wavelength tunable lasers and multi-wavelength laser arrays are perfect candidates for the WDM system.
The first report of a wavelength tunable laser was realized using the DBR structure[37]. A wavelength tuning range of 100 nm was demonstrated using super-structure-grating DBR and SGDBR lasers[38,39]. For the WDM application, a multi-wavelength laser array, which has tens of single mode lasers monolithically integrated in a single chip, is highly desirable. In 1992, Pratt
With a butterfly package, we have demonstrated an
![Photograph of (a) a packaged 8×12.5 Gb/s transmitter module, (b) the small signal responses for each channel, and (c) the measured eye diagram for each channel[43].](/richHtml/col/2017/15/1/010002/img_009.jpg)
Fig. 9. Photograph of (a) a packaged transmitter module, (b) the small signal responses for each channel, and (c) the measured eye diagram for each channel[43].
In a WDM system, an integrated laser and modulator array is also preferred, since it can achieve higher data rate for each channel. In 1999, Bell Laboratories reported the first integrated wavelength selected laser source, which has 1.55 μm DFB laser array, optical amplifier, and EAM[44]. A data rate of 2.5 Gb/s for each channel was achieved. In 2014, an
![Photographs of (a) the EADFB laser array and the structure of EADFB laser and (b) the 8×50 Gb/s module and the schematic structure of the interconnection module and photograph of Ref. [45].](/richHtml/col/2017/15/1/010002/img_010.jpg)
Fig. 10. Photographs of (a) the EADFB laser array and the structure of EADFB laser and (b) the module and the schematic structure of the interconnection module and photograph of Ref. [45].
For an integrated DFB and MZM array, Infinera reported a
Narrow linewidth high power lasers are highly required in coherent optical communications. Coherent optical fiber communications were proposed in the 1980s. It benefits from the high sensitivity of the coherent receiver, which is capable of improving the unrepeated transmission distance[48]. However, coherent optical communications have cooled down since 1990, due to the high performance of the WDM system. In 2005, digital carrier-phase estimation in a coherent receiver makes a comeback in coherent optical communication because it enables us to use many spectrally efficient modulation formats. For a coherent optical fiber or space transmission, high power and narrow linewidth lasers are preferred, which are classified as the third category of semiconductor lasers. In this category, high power performance, as well as fine optical spectrum manipulating of the semiconductor lasers, is emphasized.
The linewidth of semiconductor lasers is mainly determined by two factors. One is caused by spontaneous emission coupled to the lasing mode[49], which contributes to the white frequency noise. The other one is called 1/
Narrow linewidth semiconductor lasers have been reported based on FP, DFB, DBR, and external cavity lasers[50,51]. Actually, the linewidth of the semiconductor laser can be reduced by optimizing the laser length, as well as the optical confinement factor and coupling coefficient. Moreover, the linewidth of the laser can also be narrowed by simply increasing the driving current. In this way, the modulation bandwidth, as well as the output power, can be enhanced at the same time[52]. In 1992, the first high-speed and narrow linewidth semiconductor laser was reported by Blez
By comprehensively considering the driving current, optical coupling, the cavity of the laser, and the package parameters, we reported a narrow linewidth and high-speed DFB laser with linewidth of 130 kHz and a 3 dB bandwidth of 30 GHz[27]. Figure
![(a) Lineshape of the delayed self-heterodyne signal at 70 mA[27], and (b) the linewidth measurement setup.](/richHtml/col/2017/15/1/010002/img_012.jpg)
Fig. 12. (a) Lineshape of the delayed self-heterodyne signal at 70 mA[27], and (b) the linewidth measurement setup.
Although the linewidth of the semiconductor laser can be as narrow as tens of kilohertz, it still suffers from a wavelength stability problem due to the variation of cavity length under temperature change and mechanical vibration. We also made efforts to stabilize the wavelength of narrow linewidth laser. The narrow linewidth is realized by optical feedback. Figure
![Configuration of a wavelength-stabilized narrow linewidth semiconductor laser[54].](/richHtml/col/2017/15/1/010002/img_013.jpg)
Fig. 13. Configuration of a wavelength-stabilized narrow linewidth semiconductor laser[54].
In conclusion, this Review presents a review of semiconductor lasers for optical communications. Depending on their applications, they are classified into different categories. Their working principle, development history, and future directions have been discussed. With the increase of data traffic in future optical networks, PIC devices will attract more and more attention due to their excellent performance, compact size, and low cost. Exciting progress on photonic devices will be continued.
[1]
[2]
[3]
[4]
[5]
[6]
[7]
[8]
[9]
[10]
[11]
[12]
[13]
[14]
[15]
[16]
[17]
[18]
[19]
[20]
[21]
[22]
[24]
[25]
[26]
[28]
[29]
[30]
[31]
[32]
[33]
[34]
[35]
[36]
[37]
[38]
[39]
[40]
[41]
[42]
[43]
[45]
[46]
[47]
[48]
[49]
[50]
[51]
[52]
[54]
Ninghua Zhu. Semiconductor lasers for high-speed information technologies (Invited Paper)[J]. Chinese Optics Letters, 2017, 15(1): 010002.