Multi-gigahertz laser generation based on monolithic ridge waveguide and embedded copper nanoparticles
Download: 701次
1. Introduction
In the past few years, dielectric waveguide lasers have been well investigated by researchers to realize diverse applications in a number of areas[1–
2. Experiment
The Nd:YAG crystal was used in this work, which was cut with dimensions of [Fig. 1(a)]. The sample was implanted with ions with energy of 100 keV and fluence of [Fig. 1(b)]. During the ion implantation process, when the implantation dose is high and the concentration is above the solubility limit, the implanted impurity atoms will aggregate to form NPs. After that, C ions () with fluence of and energy of 15 MeV are irradiated into the sample, forming the planar optical waveguide with a thickness of beneath the Nd:YAG crystal surface, as shown in Fig. 1(c). Finally, a rotating diamond blade with high speed (2000 r/min) was employed to fabricate the ridge optical waveguide with a width of 23.5 µm by manufacturing parallel air grooves on the planar waveguide surface. The corresponding schematic diagram is depicted in Fig. 1(d).
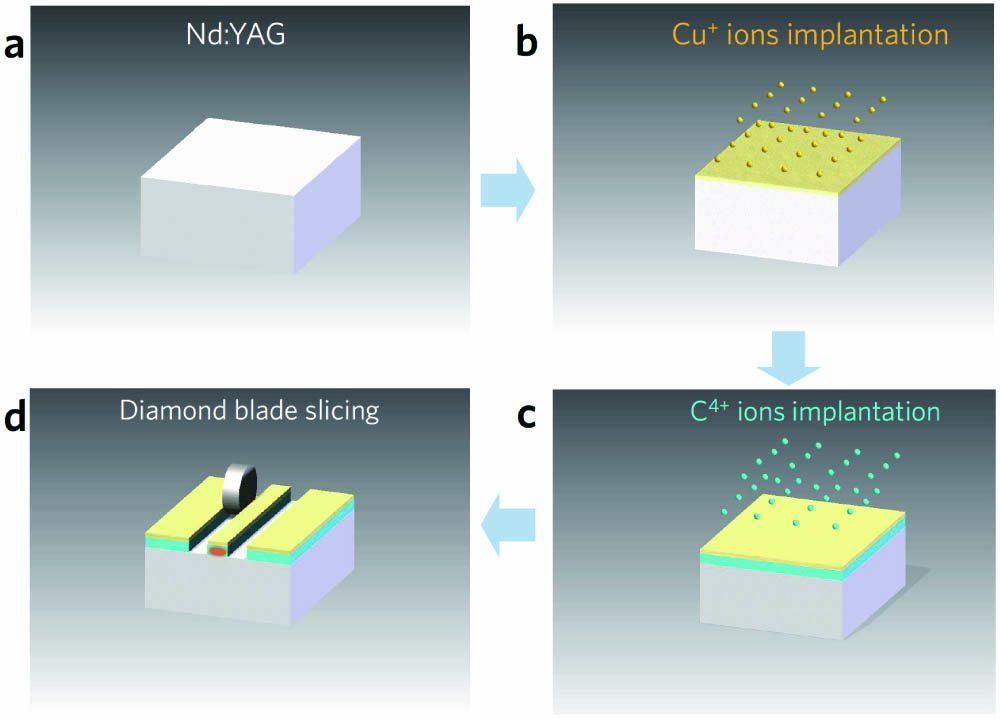
Fig. 1. Schematic of the sample fabrication. (a) Pure Nd:YAG. (b) ions implantation. (c) ions irradiation. (d) Diamond blade slicing.
Figure 2(a) shows the transmission electron microscopy (TEM) image of Cu NPs embedded YAG crystal. As indicated, the synthesized Cu NPs are mainly distributed at the depth between 50 nm and 125 nm under the surface of the sample. The element mapping image of the sample is shown in Fig. 2(b), which further confirms the formation of Cu NPs in the YAG crystal. Figure 2(c) depicts the high-resolution TEM (HRTEM) image. The interplanar spacing () of a single NP is calculated to be 0.21 nm, as depicted in the inset, which is well consistent with the crystal orientation (200) of Cu from a standard data card (PDF#040836). The diameter distribution of synthesized Cu NPs is displayed in Fig. 2(d), and the main diameter is 2.16 nm.
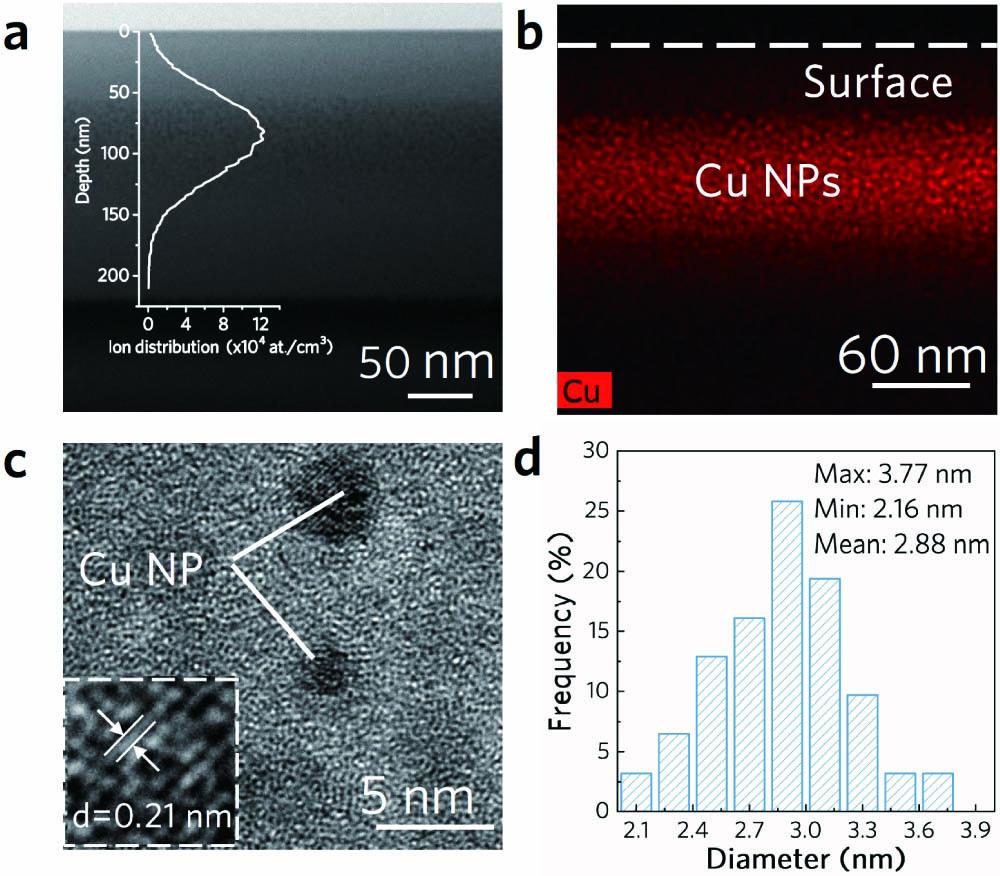
Fig. 2. (a) TEM image of the sample and projection distribution of implanted ions. (b) Cu element distribution mapping. (c) HRTEM image. (d) Diameter distribution of NPs.
3. Results and Discussion
The linear optical absorption of the Cu NPs embedded in the Nd:YAG waveguide is measured and simulated simultaneously. As indicated in Fig. 3(a), the LSPR absorption of Cu NPs shows a broad band with the peak position located at 592 nm. The inset shows the picture of the sample. It can be seen that there are a few parallel air grooves on the sample surface, which are constructed by diamond blade slicing for ridge waveguide fabrication. The absorption spectrum can be simulated by the Mie theory: where represents the volume fraction of metal (). is the wavelength of light in vacuum. and denote the complex dielectric constants of insulator and metal, respectively[37]. The simulation result is depicted in Fig. 3(b). The simulated LSPR peak of Cu NPs embedded in Nd:YAG is at 590 nm, which is consistent with the experimental result.

Fig. 3. (a) Measured linear optical absorption spectrum. Insert is the picture of the sample. (b) Calculated linear optical absorption spectrum.
The open-aperture (OA) Z-scan system operated at 1030 nm is used to measure the ultrafast nonlinear optical absorption of samples, and the corresponding schematic diagram is depicted in Fig. 4(a). The experiment was carried out using a mode-locked femtosecond laser with the repetition rate of 1 kHz and pulse width of 340 fs. The beam waist radius at the focus is measured to be . As shown in Fig. 4(b), there is no obvious nonlinear optical response for the sample without ions implantation at 1030 nm. However, saturable absorption is revealed for the sample with Cu NPs (Cu-C-Nd:YAG), which indicates significant potential in photonic devices. The saturation intensity () can be obtained by fitting the nonlinear optical response curve by the equation[38]where is the linear absorption coefficient, and is the laser intensity at different positions (). The saturation intensity is calculated to be at energy of 50 nJ, and the modulation depth is 1.5%.
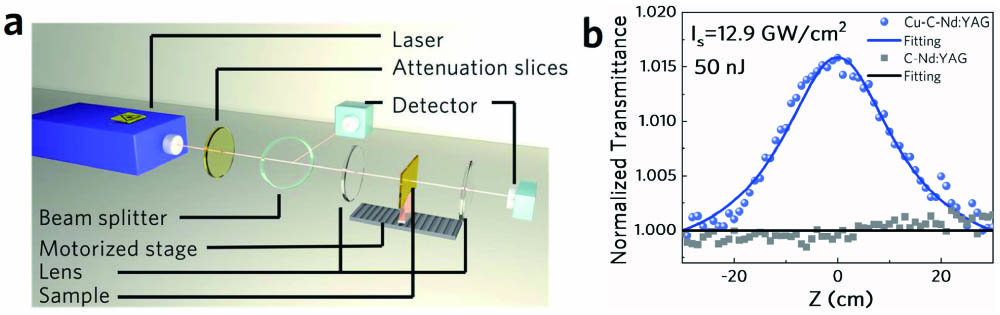
Fig. 4. (a) Schematic of OA Z-scan system. (b) The nonlinear optical response of samples under the excitation of 340 fs pulses at 1030 nm.
Based on the saturable absorption of Cu NPs, the implanted layer can be regarded as SAs and integrated on the dielectric optical waveguide. Utilizing the evanescent field coupling effect, mode-locking operation can be implemented in a tiny volume without an additional modulator. As shown in Fig. 5(a), an 808 nm continuous wave (CW) laser is coupled into the waveguide for the excitation of photons at 1064 nm. With the oscillation of photons in the laser cavity, the CW laser at 1064 nm is generated and propagated along the axis according to the total internal reflection. During the process, some photons will tunnel the potential barrier and propagate along interface between the NP layer and waveguide layer with certain distance (i.e., Goos–Hanchen shift)[39], resulting in the formation of evanescent waves. Therefore, the Cu NPs can interact with the evanescent wave sufficiently and realize the modulation of a 1064 nm CW laser for mode-locked layer generation. The evanescent field coupling effect can be demonstrated by the near-field distribution simulated by the COMSOL Multiphysics® software, as depicted in Figs. 5(b) and 5(c). It is obvious that the near-field intensity is decreased by one-order magnitude after implantation of Cu NPs. The evanescent wave is absorbed by the NPs due to the LSPR induced saturable absorption, which indicates the potential for integration of NPs and dielectric waveguide.

Fig. 5. (a) Schematic of evanescent field coupling effect. (b) Near-field distribution of the sample without Cu NPs. (c) Near-field distribution of the sample with Cu NPs.
The end-face coupling system was used to implement the 1 µm mode-locked pulsed laser generation with the pump laser at 808 nm. The laser cavity was formed by input mirror M1 (transmission of 98% at 808 nm and reflectivity of 99% at 1064 nm) and output mirror M2 (transmission of 90% at 1064 nm). The generated pulsed laser was collected and displayed by a digital oscilloscope. Figure 6(a) depicts the output power of the generated mode-locked pulsed laser as a function of the launched power. As shown, the laser threshold is 214 mW. As the launched power increases linearly to 836 mW, the output power is up to 58 mW. The emission spectrum of the output waveguide laser is displayed in Fig. 6(b), which centers at 1064 nm and corresponds to the main emission line of the ion transition. The measured near-field modal profile of the ridge waveguide is shown in the inset. Figure 6(c) indicates the single Q-switched envelope on the nanosecond (20 ns/div) time scale under the launched power of 450 mW. Figure 6(d) shows the measured mode-locked pulse train on the time scale of 400 ps/div, and the pulse duration of a single pulse is as short as 24.8 ps. The fundamental repetition rate is 7.8 GHz, and the signal-to-noise ratio is up to 47.8 dB, indicating excellent mode-locking stability. The waveguide loss was calculated by the following equation: where and represent in-coupled power and output power, respectively. The waveguide loss at the TM polarization is as low as 0.68 dB, which suggests superior waveguide properties at 1064 nm.
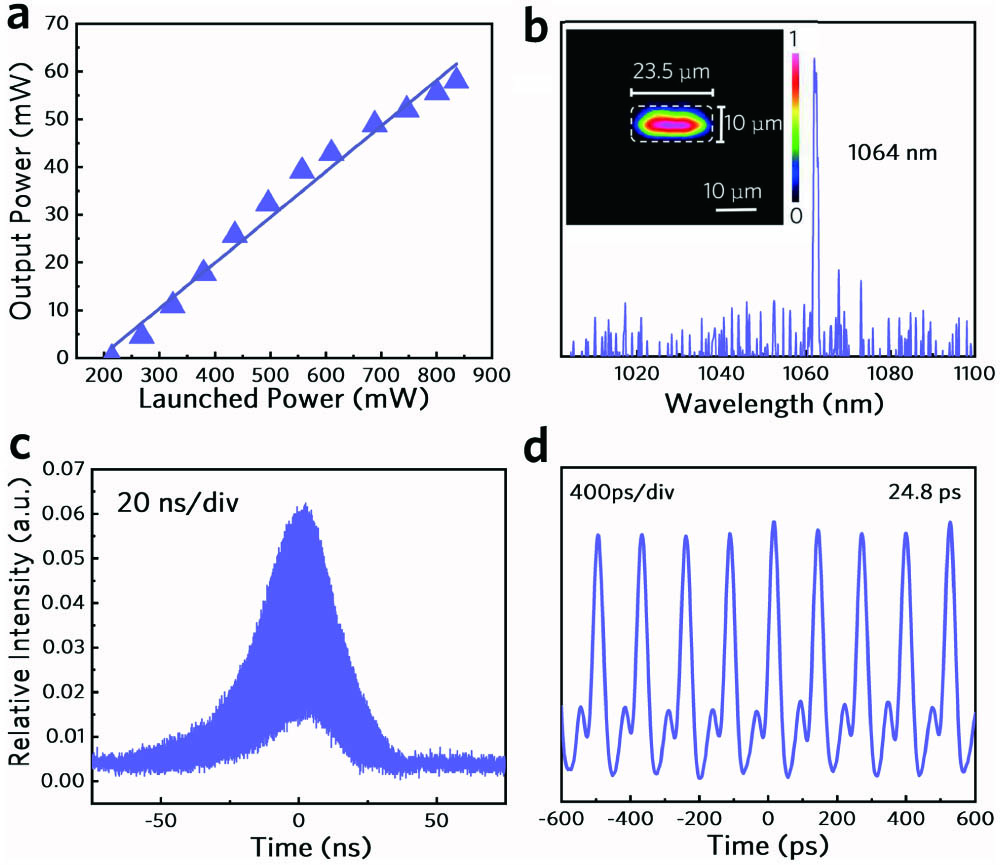
Fig. 6. (a) Output power as a function of the launched power. (b) The emission spectrum of the output waveguide laser. Inset is the near-field modal profile. (c) Single Q-switched envelope on the nanosecond time scale under the launched power of 450 mW. (d) Measured mode-locked pulse train.
4. Conclusions
In conclusion, we report on a novel monolithic waveguide chip with embedded Cu NPs on the Nd:YAG crystal platform by ions irradiation and diamond saw dicing. Excellent waveguiding properties with polarization dependence are measured by the end-face coupling system and indicate significant potential as monolithic photonic devices for laser generation. The linear and nonlinear optical responses of the monolithic waveguide chip are investigated. The LSPR peak is located at 592 nm, and the obvious saturable absorption is obtained at 1030 nm, which indicates significant potential as an SA for Q-switched mode-locked laser generation. The saturation intensity is calculated to be at energy of 50 nJ, and the modulation depth is 1.5%. In addition, the near-field distribution reveals the close interaction between NPs and the waveguide through the evanescent wave. Finally, as a potential application, a stable Q-switched mode-locked laser at 1 µm is achieved based on the evanescent coupling effect in the compact monolithic waveguide chip, reaching a repetition rate of 7.8 GHz and pulse duration of 24.8 ps. This work opens the way to realize high-performance laser generation based on the Cu NPs embedded waveguide chip.
[13] C. Pang, R. Li, Y. Zhang, Z. Li, N. Dong, L. Wu, H. Yu, J. Wang, F. Ren, F. Chen. Tailoring optical nonlinearities of LiNbO3 crystals by plasmonic silver nanoparticles for broadband saturable absorbers. Opt. Express, 2018, 26: 1276.
[15] W. Zhao, G. W. Chen, W. L. Li, G. M. Wang, C. Zeng. All-fiber saturable absorbers for ultrafast fiber lasers. IEEE Photon. J., 2019, 11: 7104019.
[26] Y. Zhang, D. Lu, H. Yu, H. Zhang. Low-dimensional saturable absorbers in the visible spectral region. Adv. Opt. Mater., 2019, 7: 1800357.
[31] B. Y. Guan, L. Yu, X. Wang, S. Y. Song, X. W. Lou. Formation of onion-like NiCo2S4 particles via sequential ion-exchange for hybrid supercapacitors. Adv. Mater., 2017, 29: 1605051.
[32] A. L. Stepanov. Nonlinear optical properties of implanted metal nanoparticles in various transparent matrixes: a review. Rev. Adv. Mater. Sci., 2011, 27: 115.
[33]
Chi Pang, Rang Li, Ziqi Li, Ningning Dong, Jun Wang, Feng Ren, Feng Chen. Multi-gigahertz laser generation based on monolithic ridge waveguide and embedded copper nanoparticles[J]. Chinese Optics Letters, 2021, 19(2): 021301.