Great enhancement of image details with high fidelity in a scintillator imager using an optical coding method
Download: 634次
1. INTRODUCTION
In indirect X-ray imaging technology, scintillators are employed to convert X rays to visible light and are extensively used in medicine, materials, life sciences, nondestructive testing, and for industrial inspections. The scintillator imagers coupled with microscope lenses [1,2] possess high-resolution and strong radiation-resistance characteristics. They are extensively used in various high-performance imaging experiments based on high-brightness synchrotron radiation sources, especially in biomedical research. A micrometer-level resolution can be achieved by this system. However, the images are often not as clear as expected in the lens-coupled scintillator imagers. The image details are often mixed with high-frequency noise. Increasing the X-ray luminous flux is costly and hardly helps to solve the problem. At present, the research and development efforts on new scintillators are still focused on the improvement of the fluorescence conversion efficiency. It has also been noticed that the scintillator has a high refractive index in the range of 1.8 to 2.5 [3] for its visible fluorescence, which thus causes nearly 92% of the stimulated visible photons to be lost on the exit surface. A seemingly possible solution to this problem may involve the extraction of the maximum number of photons [4,5]. However, even this approach does not help improve the quality of the image [6].
We found that a high refractive index of the scintillator significantly degrades the pupil function and thus weakens the extensive broadband high-frequency information, which has a major impact on the image quality. Therefore, we intend to use optical image coding methods to enhance this information that is critical to the image characteristics.
The optical encoding method has been applied to convert evanescent waves that carry high-frequency information into transmission waves in far-field, super-resolution imaging systems with coherent illuminations. For example, Gustafsson [7] developed structured light illumination microscopy to shift the spatial frequencies of the image. A multiple-illumination field structure was then constructed to provide sufficient phase information for image decoding. However, it is difficult to apply this technique in our incoherent imaging system in which (i) the source’s phase information is lost, which is necessary for decoding, and (ii) a nanostructure with a very high aspect ratio is required to block hard X rays. This nanostructure is difficult to fabricate. Zhang et al. [8] introduced a far-field optical superlens to considerably enhance the evanescent waves from an object and convert them into propagating waves in the far field. The surface plasmon polariton (SPP) employed in this scheme [9] mainly responded to infrared and visible lights in the red part of the spectrum, while the cerium-doped yttrium aluminum garnet (YAG:Ce) used in our system emitted light with wavelengths in the range of 500–600 nm. More importantly, compared with the above super-resolution techniques, our research was focused on the quantity of high-frequency and medium-high-frequency signals to improve the imaging quality in high-fidelity applications instead of extending the upper frequency limit.
Unlike the above applications on super-resoution, a new optical image encoding–decoding technique is now proposed to enhance the middle-high-frequency and high-frequency broadband information, to break through the bottleneck of the high-resolution lens-coupled X-ray scintillator imager. In this technique, a two-dimensional (2D) high-density array is used as an encoder to extract broadband high-frequency information instead of photons. The high-density array with periods of 300 nm was designed and covered on a (YAG:Ce) scintillator film by electron beam lithography (EBL). The image obtained by a scientific complementary metal-oxide semiconductor (sCMOS) camera was finally decoded with an iterative algorithm. We refer to this technique as the high-spatial-frequency spectrum enhanced reconstruction (HSFER) method. It will be shown that upon use of this technique, the signal-to-noise ratio (SNR) in the middle-high-frequency region of an incoherent image can be increased by 1 order or more. Fidelity was maintained during the imaging process. Another possible application might be expected to lie in low-dose medical diagnostics [10,11]. With a synchrotron-radiation X-ray source [12,13], the fast imaging capability of this technique can also provide a good opportunity to study the dynamics of various samples [14,15].
2. THEORY
2.1 A. Principle
A new encoding–decoding imaging method, commonly referred to as HSFER, is proposed herein (Fig.
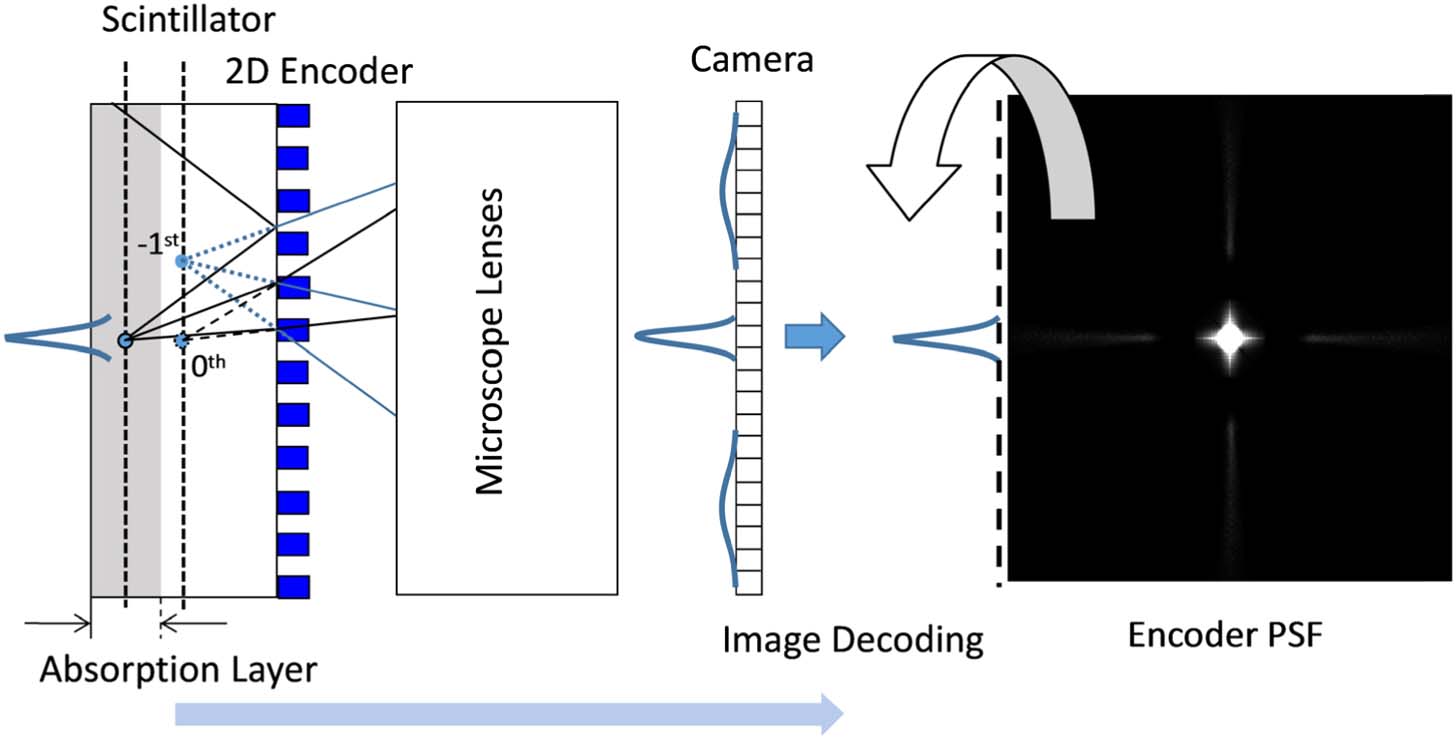
Fig. 1. Schematic of an X-ray scintillator imager based on the use of the proposed high-spatial-frequency spectrum enhanced reconstruction (HSFER) method. A two-dimensional (2D) encoder is used to extract the middle-high-frequency and high-frequency components of the image generated in the scintillator. The image is decoded by a PSF/OTF of the encoder.
The high-frequency information in an image determines the image characteristics. In the case of the YAG:Ce scintillator imager, the refractive index is as high as at a wavelength of 550 nm. The reflectivity increases abruptly as a function of the angle. The diffraction limitation has profound effects. Therefore, severe loss of high-frequency information occurs, and the image quality is degraded. Most of the useful information carried by the waves with large emission angles, especially the waves that are totally reflected, is absent in a far-field imaging system [16].
The starting point of our encoding–decoding imaging theory is the simple coherent imaging case. We assume the existence of an object field produced by illumination in the presence of coherent waves. In a practical imaging system, the system’s point spread function (PSF) accounts for the image degradation factors. Therefore, the object function can be recovered as follows: where denotes a degraded image, represents the convolution operation, and is the system’s PSF. The corresponding incoherent imaging process can be described as To improve imaging quality, an optical encoding function can be integrated into the system described by Eq. (
Assuming that the camera noise is negligible and only image noise is considered, could be replaced by . The corresponding Fourier transformed quantities and can be obtained with the use of Eq. (
Many scintillators have a broad fluorescence spectrum. Correspondingly, the case of multiple wavelengths is considered in this case. The aforementioned object field and image field are independent of the fluorescence wavelength, and the optical imaging results with different wavelengths can be accumulated directly. Owing to the different diffraction angles, the pupil functions that correspond to different wavelengths are also different from each other, i.e., . The information delivered by different wavelengths of light can complement each other, and it can thereby expand the spatial frequency spectrum of the image. The imaging process with a light source having a continuous spectrum is described by where is the bandwidth of the wavelength spectrum. If we define and , Eq. (
For the image decoding process, it is easy to know that the overall difference between the image with and without HSFER is not large. The second term on the right side of Eq. (
A 20 μm pinhole was employed to obtain this “encoder OTF” . The imaging experiment was carried out on the beam line BL09B of the Shanghai Synchrotron Radiation Facility (SSRF). The results were summed up to achieve a high SNR. Then was calculated by an iteration version of Eq. (
2.2 B. Key Parameters of the 2D Encoder
We still begin this subsection from the simple coherent imaging case. The 2D encoder lattice structure will affect the diffraction in the same lattice orientation. For simplicity, a one-dimensional imager is analyzed here. The spatial frequency spectrum of an image can be filtered by the system’s pupil function. There are several factors that may affect this pupil function, including (i) numerical aperture (), which is set to 0.4 in our optical system, i.e., acceptance angle is 12.8° in the scintillator, (ii) total reflection angle of the scintillator, which is equal to 33.7° in the case of the YAG:Ce scintillator, and (iii) the nanostructure of the 2D encoder on the exit surface of the scintillator. The upper limit of the spatial frequency is still determined by the N.A. of the system. However, due to the high refractive index of the scintillator, the reflectivity is increased rapidly as the incident angle increased. And the middle-high-frequency and high-frequency information decays quickly.
The forward diffracted waves from the object are first incident on the exit surface of the scintillator and are then diffracted again by this 2D encoder. This process can be described by the grating equation as follows: where is the reciprocal lattice vector of the photonic crystal, is the grating period, is the diffraction order, is the fluorescence wavelength in vacuum, is the refractive index of the scintillator, and is the incident angle on the 2D encoder surface.
Even though the period of the structure is smaller than the wavelength, the grating equation [Eq. (

Fig. 2. (a) Areas below the dashed, dotted, and solid curves represent the parameter zones that allow the st-order diffraction to occur for the two-dimensional (2D) encoder periods of 600, 450, and 300 nm, respectively. This shows that the encoder with a 300 nm period can yield a maximum suppression of the -order diffraction compared with the other two encoders. In fact, no st-order diffraction occurs with a 300 nm period in FDTD simulations. (b) The green area shows the parameter zone that allows the st-order diffracted waves to be accepted by the optical system, whereby the encoder with the 300 nm period is selected. The angle 33.0° represents the total reflection angle. The FDTD simulation results of the transverse electric (TE) and transverse magnetic (TM) modes are also shown here. (c) MTFs are used for the evaluation of the performance of this system. The thick curve represents the MTF of the case with the 2D encoder, while the thin one represents the case without the 2D encoder. Obviously, the spectrum is extended considerably by the encoder. The value is the cutoff frequency determined by the equivalent camera pixel size, which is equal to in our experiment. The green area indicates the extra high-frequency information received from the encoder.
The st-order diffracted wave from a grating with a subwavelength period can preferentially carry high-frequency image information because the incident waves of the large angles carrying high-frequency information are more likely to satisfy the diffraction conditions and thus can be transformed into transmission waves. Meanwhile, the positive-order diffractions might be suppressed by this grating. Figures
The above analysis is based on the coherent imaging case in which one can calculate the simplified coherent transfer function (CTF) of the system based on the well-known coherent imaging theory [17]. However, in our system, the lights were emitted by incoherent luminescence centers within the scintillator. The OTF, which is the autocorrelation function of the aforementioned CTF, is calculated and shown in Fig.
3. EXPERIMENTAL SETUP
A 2D encoder with a 300 nm period and a duty cycle of 60% in a square lattice was fabricated by electron beam lithography (EBL) [18,19] and inductively coupled plasma (ICP) etching [20]. Figure

Fig. 3. Experimental setup with the HSFER imager. The fluorescent pattern produced by the X rays through the sample was first encoded by a 2D encoder, then imaged by the camera, and finally decoded by an iteration method. The inset upper-left subfigure shows the scanning electron micrograph of the 2D encoder: a YAG:Ce film covered with a 2D array.
The Richardson–Lucy deconvolution algorithm was employed in image decoding. The calculation parameters were carefully adjusted to avoid the overshoot phenomenon. Otherwise, false high-frequency information could be introduced.
4. EXPERIMENTAL RESULTS
Figure
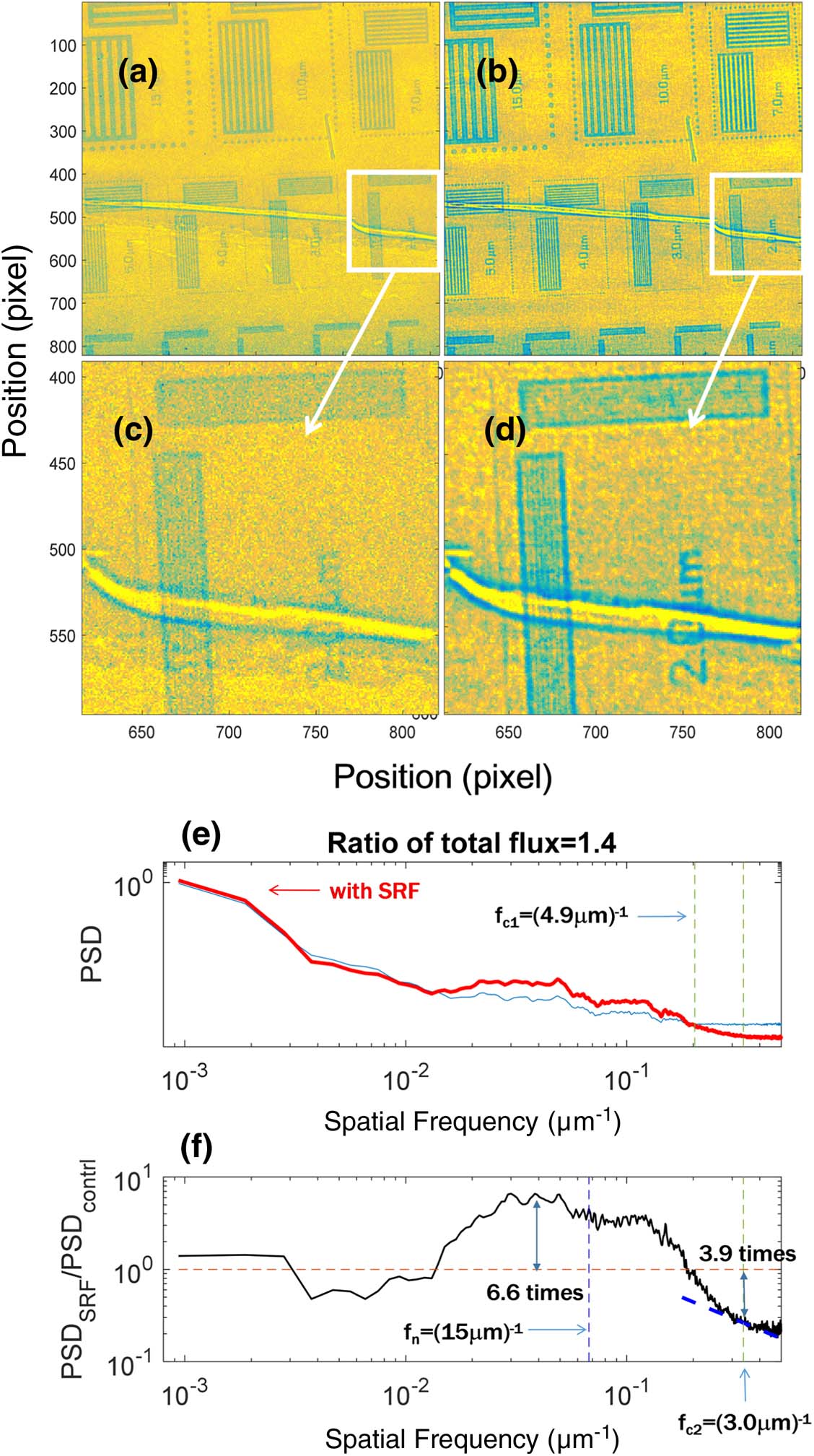
Fig. 4. Radiographs of the resolution chart obtained with a 3 s exposure (the dose is ). (a), (b) Imaging results for a resolution chart without and with the HSFER method, respectively. (c), (d) Magnified views of the regions of interest in (a) and (b), respectively. (e) PSDs of the images in (a) and (b), whereby the red and the blue curves represent the cases with and without the HSFER, respectively, and is the cutoff frequency for the thin curve. (f) Normalized PSD with respect to the one without HSFER, thus showing that the cutoff frequency is extended to . The is the spatial frequency at which the average effect of the deconvolution algorithm begins to work. The dashed line describes the denoising effect for frequencies much higher than .
To investigate the imaging at low-exposure doses with the HSFER method, the resolution chart was imaged with a 0.5 s exposure. Obviously, the HSFER results can visualize an extensive number of sample details that are almost indistinguishable in the non-HSFER images. The quality of the middle-high-frequency part is outstanding, and the SNR is improved by approximately 52 times, as shown in Fig.

Fig. 5. Radiographs of the resolution chart obtained with a 0.5 s exposure (the dose is ). (a), (b) Imaging results without and with the HSFER method, respectively. (c), (d) Magnified views of the regions of interest in (a) and (b), respectively. (e) PSDs of the images in (a) and (b), whereby the red and blue curves represent the cases with and without the HSFER, respectively. (f) PSDs with respect to the case at which the HSFER was not used.
We applied the HSFER technique to image a practical biological sample, i.e., the gill region in a dehydrated zebrafish. The parallel secondary lamellae on both sides of each gill filament denote the respiratory sites of gas exchange [22]. These can be observed from the ambiguous signals in the radiograph [Fig.
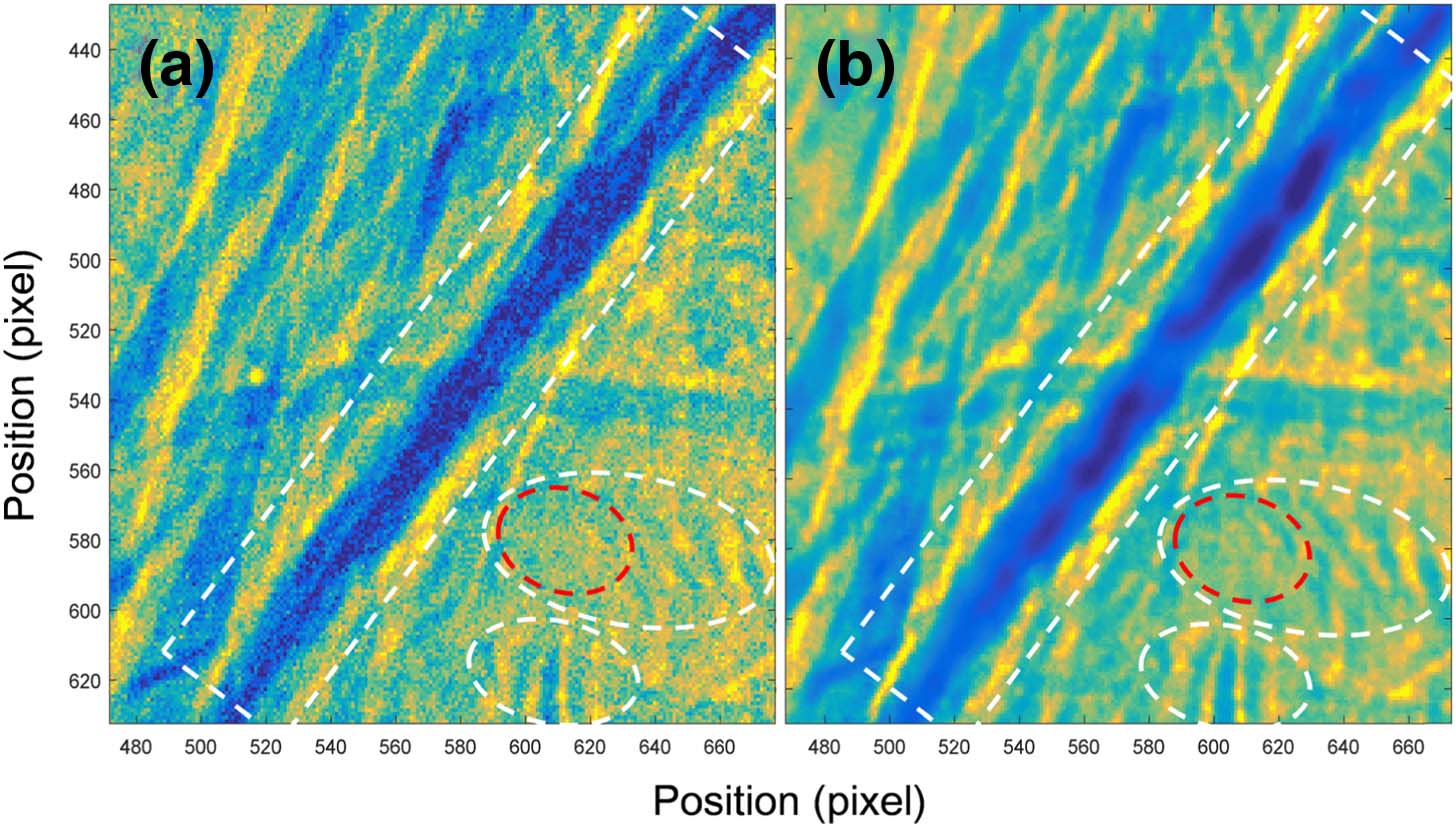
Fig. 6. Radiographs of the gill of a dehydrated zebrafish following an exposure of 1 s (the dose is ). More detailed structures in the regions of interest can be found in (b) the HSFER image compared with that in (a) the conventional image.
5. CONCLUSIONS
In summary, a new X-ray indirect imaging technology, known as the HSFER method, was proposed to retain the image details in lens-coupled scintillator imagers. This method was demonstrated successfully based on (i) resolution chart imaging tests and (ii) a practical biological specimen imaging experiment. This method is essentially applicable to spatial incoherent imaging with broadband sources. In a scintillator imager using the HSFER method, the optical information was encoded by a 2D high-density grating and decoded with an iteration method. During this process, the middle-high-frequency and high-frequency information was exploited and led to high-SNR images with high fidelity, even in the case of low-dose X-ray irradiation. Furthermore, the noise equivalent resolution was improved. This method is expected to be applicable with various X-ray sources, such as large synchrotron radiation devices and desktop X-ray tubes, and is expected to enable low-dose and high-quality imaging.
6 Acknowledgment
Acknowledgment. The authors are grateful for the support of SSRF-BL08U1B beamline for sample preparation and the help from SSRF-BL08U1A. Special thanks are attributed to Wencong Zhao, Mei Chen, and Huaina Yu for their preliminary work related to this research. Thanks are also extended to You He and Zijian Xu for their help.
Article Outline
Huijuan Xia, Yanqing Wu, Lei Zhang, Yuanhe Sun, Zhongyang Wang, Renzhong Tai. Great enhancement of image details with high fidelity in a scintillator imager using an optical coding method[J]. Photonics Research, 2020, 8(7): 07001079.