Reducing the mode-mismatch noises in atom–light interactions via optimization of the temporal waveform
Download: 616次
1. INTRODUCTION
Atom–light interactions are crucial for precision measurements and quantum information processing based on the atomic systems including an atomic quantum repeater, atomic magnetometer, and atomic interferometers [1
As a promising candidate, Raman processes have been widely used in atomic quantum information processing, such as Duan-Lukin-Cirac-Zoller (DLCZ) quantum repeaters [18] and Raman quantum memory [19], and also in atomic precision measurements, e.g., the beam-splitting and recombination processes for atomic interferometers [20,21] and atom-light hybrid interferometers [17]. In Raman processes, the excess noise introduced by the spatial multimode is generally concerned. Noise reduction via spatial mode cleaning has been demonstrated [22,23]. Recently, the temporal modes of optical fields have been extensively studied to increase information capacity [24,25], to improve entanglement of two-mode squeezed light [26,27], and to give a new quantum information proposal [28]. The noise reduction in Raman scattering should be achieved by temporal mode cleaning in atom light interaction, which has not yet been reported.
In this paper, we experimentally demonstrate noise reduction by optimization of the temporal waveform. We achieve the optimized temporal mode (TM) of input Stokes light in the SRS by the iteration method. The results show that whatever the initial input Stokes waveform is, the final optimized TMs of Stokes light are the same by iterative optimization with a fixed atomic system and a certain strong Raman pump pulse. Furthermore, the effect of the TM on the interaction noises is carefully investigated. We measure the noise fluctuation of the output Stokes field and the intensity difference between the Stokes field and the accompanied atomic spin wave, and we compare the variance data in optimized and nonoptimal cases. The intensity fluctuation of the generated Stokes field and the intensity difference decrease by 4.3 dB and 3.1 dB, respectively. Such a notable noise reduction of the Stokes field in SRS should be beneficial to improve the precision of gravity and angular velocity based on atom interferometers. Furthermore, reducing the noise fluctuation of the intensity difference indicates taking a step further toward the generation of an atom–light squeezed state, which should have a wider application in quantum information processing.
2. TEMPORAL MODE OPTIMIZATION
2.1 A. Experimental Setup
The schematic of the experimental setup to perform the SRS including the temporal waveform iteration optimizing process is shown in Fig.

Fig. 1. (a) Schematic of the experimental setup. PBS, polarized beam splitter; HWP, half-wave plate; EOM, electro-optic modulator; AOM, acousto-optic modulator; PD, photodetector; AWG, arbitrary wave generator; DPO, digital phosphor oscilloscope. (b) Energy levels of the atom and the frequencies of the lasers: , hyperfine levels ; , hyperfine levels and ; , the Raman pump field; , the initial input Stokes seed; , the output Stokes field via SRS; OP, the optical pumping; , the atomic spin wave; , the read field; , the output anti-Stokes field by the reading process.
2.2 B. Iterative Optimization
The interaction Hamiltonian for the SRS is well known [18,29]: , where is the interaction coupling coefficient. The related input-output relationship can be written as , in which and are gain factors and satisfy . In principle, the input Stokes seed () and the amplified output Stokes field () of the SRS can be expanded using a complete normalized orthogonal basis set of the temporal mode (TM) [30]: where is the Stokes annihilation operator for the th-order TM, and and are the corresponding probability amplitudes and satisfy . To simplify the expression, we redefine the optical temporal mode operator , whose commutation relation satisfies . The above basis set is related to the Raman pump field [31]. The temporal waveform of the initial Stokes field can be decomposed using with the corresponding ratio coefficients . Then TMs of different orders are amplified with the corresponding gain factor , which are related with the atomic optical depth, single-photon detuning, and the intensity of the Raman pump field. The final output Stokes field is the sum of all amplified TMs with the corresponding ratio coefficients . It is hard to obtain the exact analytical form of the TM basis set. However, the gain factor corresponding to the lower-order TM is usually larger than that for the higher-order TM: [32]. So after each SRS process, we should get a higher proportion of lower-order TMs and a lower proportion of higher-order TMs in compared with in . Based on this, we design an iteration procedure to get the final optimized TM of the SRS.
The iterative optimization [33] is operated as shown in Fig.
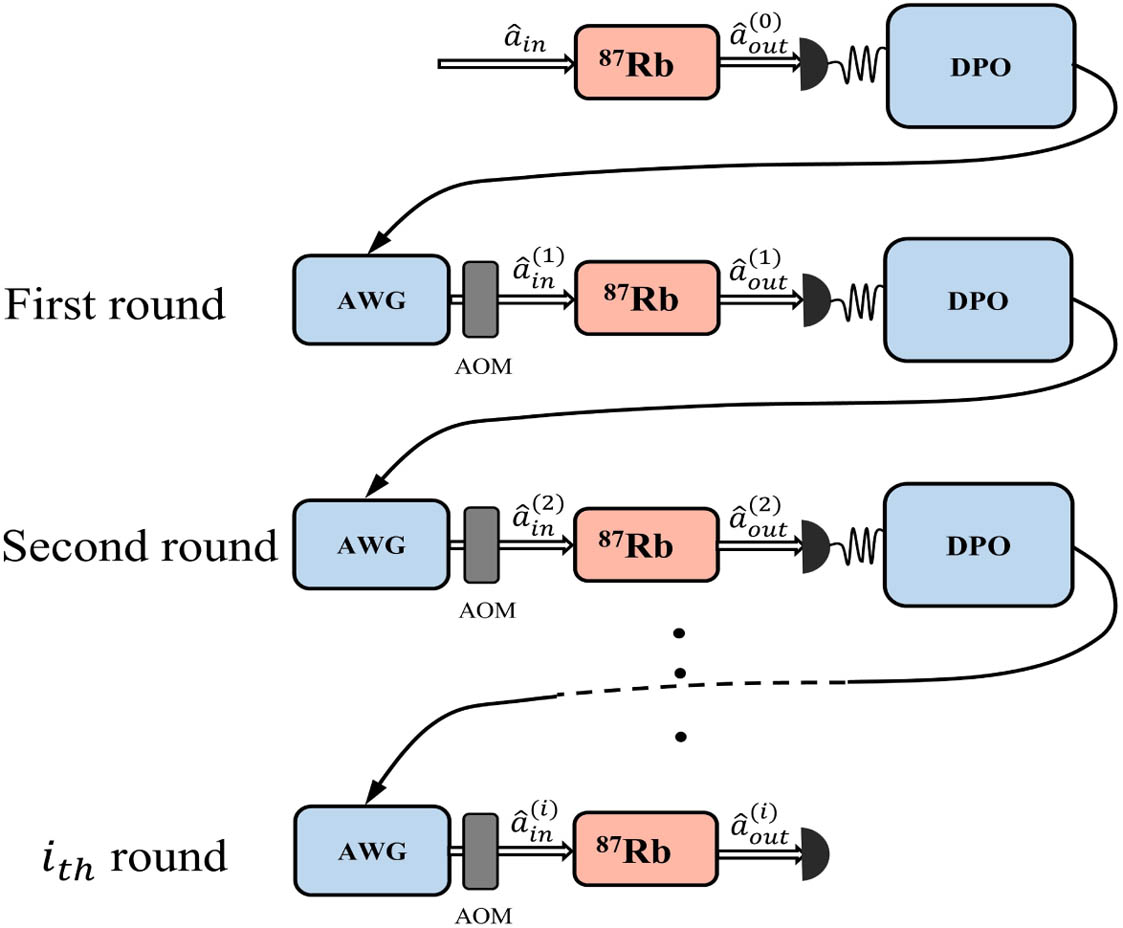
Fig. 2. Iteration diagram. are normalized initially input Stokes seeds; are output signals by SRS, corresponding to the th iteration.
We utilize the experimental setup shown in Fig.

Fig. 3. (a) and (b) is the initially input seed waveform and is the corresponding output signal of SRS. is the output signal of SRS in the first round, is stable waveform after several iteration rounds. (c) Pink circles and green solid triangles are corresponding to different in (a) and (b), respectively.
In this paper, only the first TM is achieved through the iterative procedure. But in principle, higher-order TMs can also be picked up by subtracting the lower-order TMs in the input seed following the Gram–Schmidt process and repeating the iterative procedure. The related work has been reported in Ref. [32], where the first three orders of mutually orthogonal TMs have been experimentally characterized. Here we achieve the optimal Stokes shape with given Raman pump shape. In principle, the optimal Stokes shape is related with the Raman pump because the output Stokes field depends on the amplitude of the Raman pump in theory [31].
3. NOISE PERFORMANCE
3.1 A. Stokes Noises
Just like any other parametric amplified processes, in SRS, the gain of the Stokes field is also along with some inevitable extra noises, including quantum noises and technical noises. There has been much previous research [3436" target="_self" style="display: inline;">–
As described above, under given experimental conditions, or stands for the optimized TM of SRS, which should correspond to the larger gain factor as a result of the better mode match between the interaction system and the input Stokes seed [32]. As a comparison, the input seed of the arbitrary temporal waveform consists of different order TMs that are orthogonal with each other. Since the stimulated amplification of each order by SRS is not relevant to the other orders, and the final output signal is the sum of all modes, it may cause significant intensity fluctuation of the output signal to limit the measurement precision. For example, for atomic interferometers using SRS as beam-splitting and recombination processes, the intensity fluctuation of the output signal is certain to cause the instability of the wave-splitting ratio. In the respect of quantum noise alone, the larger gain effect also brings in more associated noises [37]. However, as shown below, our experimental results show that the output Stokes noise is actually suppressed in the case of an optimized Stokes seed corresponding to the larger gain factor compared with the nonoptimal case. So the 4.3 dB improvement in Fig.

Fig. 4. (a) and (b) The different energy fluctuations of and of during many measurement times. (c) is the average energy of the output Stokes field. Black square and red triangle curves are respective for and both with 100 ns long square- pulse.
To test the noise reducing effect of temporal mode selection, we experimentally measure the intensity variance of with the nonoptimal square Stokes seed and with the optimized TM obtained in the iteration procedure. We record more than sets of single output signal using an oscilloscope, and then obtain the pulse energy data by integrating each shot. The data in cases of and are given in Figs.
In atom interferometers, the beam splitting for the atomic waves is operated by an SRS process. The intensity fluctuation of the amplified Stokes field causes the number fluctuation of the atomic waves, given the general expression of the phase sensitivity [38] . So in principle, the noise reduction of 4.3 dB ( times) in the output Stokes field will result in about 2.2 dB ( times) improvement of the phase sensitivity.
3.2 B. Intensity Difference Noises
In Raman scattering, generation of always accompanies with atomic spin wave as shown in Fig.
Different from the Stokes noise discussed in Section
To experimentally measure the intensity difference, a strong 100 ns long read pulse () is sent into the atomic system after the generation of . The frequency of the read laser is red detuned 1.0 GHz from the atomic transition . The read pulse retrieves the atomic spin wave back into an optical signal . In an atomic vapor cell, the atomic decoherence is mainly due to atoms flying out of the interaction region. To retrieve an as large as possible, the pulse is turned on just for 100 ns. The delay between and is 50 ns. and pass the same path. We record and in each cycle. The measured retrieved efficiency is 70%. , are the average energies of , , respectively.
Under the certain and , we record sets of and , and we take in each cycle as the intensity difference between and . Then a series of the variance of , can be acquired by changing the intensity of the input seed. The results are plotted in Fig.

Fig. 5. Black square and red triangle curves are respective for and both with the square- and square- pulse acquired in the experiment.
4. CONCLUSION
In this paper, we demonstrate the reduction of mode-mismatch noises in atom–light interactions by temporal mode cleaning. The first-order temporal mode is achieved by the iteration method, which depends on the Raman pump shape whatever the input seed is. By using the optimized temporal mode, the noise fluctuation of the output signal decreases by 4.3 dB compared with the nonoptimal case. Meanwhile, the noise fluctuation of the intensity difference between the signal and atomic spin wave is reduced by 3.1 dB. Our results show that the temporal multimode, similar with the spatial multimode, also contributes significant noises into the measurements based on atomic systems. This point has always been ignored in previous research. Our results can also be applied to crystal systems [41,42] and should be helpful to improve the precision of atomic measurement and to achieve the atom–light squeezed state.
Article Outline
Xiaotian Feng, Zhifei Yu, Bing Chen, Shuying Chen, Yuan Wu, Donghui Fan, Chun-Hua Yuan, L. Q. Chen, Z. Y. Ou, Weiping Zhang. Reducing the mode-mismatch noises in atom–light interactions via optimization of the temporal waveform[J]. Photonics Research, 2020, 8(11): 11001697.