Control of the harmonic near-field distributions by an active metasurface loaded with pin diodes
1. INTRODUCTION
Metasurfaces have attracted enormous interest due to their intriguing electromagnetic (EM) responses, which bring us many fascinating physical phenomena including negative refraction [1,2], perfect lensing [3], and invisibility cloaking [4–6]. The metasurfaces usually consist of the resonant or nonresonant subwavelength elements, whose reflection/transmission coefficients can be customized at will by changing the size, shape [7], or orientation of unit cells [8–10]. Due to the elaborate arrangement of the metaatoms across the whole metasurface, the phase discontinuities can be introduced to control the propagation direction [11], polarization state [12–14], and wavefront [15–19] of EM waves. To simplify the complexity of metasurface design, coding metasurfaces [20,21] were proposed to facilitate accurate control of the EM waves based on the coding sequences of binary metaatoms. Hence, versatile functionalities of the coding metasurfaces have been demonstrated, including ultrathin planar lenses [22,23], vortex beam generations [24–26], and metasurface holograms [27–30].
However, most of these metasurfaces are passive, and their functions are fixed once they have been fabricated. To overcome these limitations, dynamic control of the EM waves was proposed based on programmable/tunable metasurfaces. These metasurfaces are usually integrated with active devices, such as positive-intrinsic-negative (PIN) diodes [31–35], varactors [36–39], and microelectro-mechanical systems [40]. However, most of the aforementioned programmable metasurfaces only focus on the control of the incident carrier wave, but seldom realize accurate manipulations of nonlinear amplitudes and phases.
In the THz and visible regimes, plenty of metasurfaces were proposed to control nonlinear wave responses, including the intensity, phase, and the polarization state [41–45]. However, the simultaneous control of the harmonic amplitudes and phases still remains a great challenge. Recently, space-time-digital metasurfaces [46–50] were proposed to solve this problem. By dynamically altering the local amplitude or phase of the surface reflectivity in a periodic manner, the metasurfaces showed powerful capabilities in the generation and manipulation of a specific order harmonic [46–50]. Furthermore, Dai
On this basis, we propose a scheme to realize the manipulation of the fundamental and high-order harmonics simultaneously via the space-time digital metasurface. A novel experimental platform for nonlinear field mapping is developed to monitor the harmonic near field distributions. As an example, we show the feasibility of achieving two symmetric beams at the fundamental frequency, and the orbital angular momentum (OAM) beams with different topological numbers at st order harmonics. The space-time digital metasurface is designed, fabricated, and measured at different harmonics. The experimental results validate the effectiveness of the theoretical analyses.
2. THEORY AND DESIGN

Fig. 4. (a) Required phase distributions of the different harmonics. (b) The corresponding far-field scattering patterns. (c), (d) Near-field amplitude and phase distributions for the st, 0th, and 1st order harmonics.
Under the monochromatic wave incidence , the reflected spectrum is composed of many harmonics [46]. is defined as the complex Fourier coefficient of the periodic reflectivity at the th order harmonic , where , , , , . and are the modulation frequency and the carrier frequency, respectively. can be written as
In this study, we consider a high-efficiency reflective metasurface with . Two states, namely 0 and 1 states, are found with the reflection phases of to be 0° and 180°, respectively. From Eqs. (2) and (3), the duty cycle of the reflection phases of is very important for the energy conversion from the fundamental wave into the different harmonics. As an example, altogether 12 time slots with the duty cycle of 1/3 are selected to get the larger amplitudes for the 0th, st, 1st order harmonics. For simplicity, the basic coding sequence is chosen as 000011111111, implying that the reflection phases are 0° in the first four time slots, and 180° in the last eight time slots. The corresponding reflection amplitude and phase spectra can be found in Fig. 2(a), with and . However, as demonstrated in Figs. 2(b) and 2(c), the phases and time delays also show great influence on the harmonic phase distributions, which is consistent with the prediction from Eq. (4). Reflection amplitudes and phases of Reflection amplitudes and phases of
From Eq. (4), the harmonic phases for the fundamental and st order harmonics are obtained as
According to Eqs. (2)–(4) when the coding sequence and period are determined, , , and are fixed. Thus, we control the phases of any two harmonics independently by choosing the proper sets of (, ), which enables us to design and realize various functionalities at different harmonics.

Fig. 3. (a) Perspective view, (b) top view, and (c) bottom view of the metaatom. (d) The simulated reflection spectra of the metaatom under the normal incidence. (e) The scattering patterns of the metaatom for the on and off states. The geometric parameters of the metaatom are , , , , , , , , , and .
The commercial software CST Microwave Studio 2019 is used to investigate the reflection spectrum of the metaatom under different working states of the PIN diode. During the simulation, the unit-cell boundaries are applied in both the and directions to incorporate the mutual coupling effect between adjacent elements. Two Floquet ports for -polarized waves are applied in the and directions.
The simulated reflection amplitudes/phases and the corresponding far-field scattering patterns are given in Figs. 2(d) and 2(e), respectively. When the PIN diode is turned from on to off, the phase difference is approximately 180° from 6.5 to 8.5 GHz, and the corresponding amplitudes are over 0.9, which means the element can work in both the 0 and 1 states under different biasing voltages. No matter the PIN diode is switched on or off, the scattering wave from the metaatom under normal incidence is always restricted on both sides of the normal direction as illustrated in Fig. 3(e), and the total scattering waves from the metasurface are the superposition of those from the composing elements. Therefore, it is easy to find that the field intensity along the normal direction of the metasurface is the largest.
To illustrate the independent manipulations of the EM waves at both the fundamental and higher-order harmonics, a space-time digital metasurface is encoded to realize the two symmetric beams at the fundamental frequency, and an OAM beam at the st order harmonics. Here, we need to remark that if we want to get an OAM beam with the topological number for the 1st order harmonic, another OAM beam with the topological number is also achieved at the st order harmonic, which can be explained from Eq. (5). When the initial phase and the parameter are fixed, it will cause different influences on the st order harmonics by changing the time delay . In other words, if the time delay is increased, the st harmonic phase is increased as well, while the 1st harmonic phase is decreased with the same degree, giving rise to the opposite topological number as a result.
The space-time digital metasurface consists of metaatoms, which are specifically divided into the eight regions [marked from to , in Fig. 4(a)] with the same coding sequences , but different combination values (, ). For the fixed coding sequences 000011111111, can be calculated as 180°, 60°, and for the 0th, st and 1st order harmonics, respectively, from Eq. (3) and Fig. 2(a). From Eq. (5), the phase at the fundamental frequency can be adjusted by the initial phase , and the different harmonic phases can be obtained by changing the time delay at the st order harmonics. (a) Required phase distributions of the different harmonics. (b) The corresponding far-field scattering patterns. (c), (d) Near-field amplitude and phase distributions for the (a) Required phase distributions of the different harmonics. (b) The corresponding far-field scattering patterns. (c), (d) Near-field amplitude and phase distributions for the
As shown in Fig. 4(a), to achieve two split beams [19–21] at the fundamental frequency, the element phases are 0° in the left half part (regions ), but 180° in the right half (regions ). In addition, to realize an OAM beam with the topological number at the 1st order harmonic, the phase distribution of is shown in Fig. 4(a), which is gradually increased by a step of 45° from region to the region . Substituting , , , , and into Eq. (5), the values of and in the eight regions are obtained, as listed in Table 1. Note that the phase profile of the st order harmonic is just opposite to that of the 1st order harmonic, as demonstrated in Table 1, leading to the OAM beams with the opposite topological numbers. Combination Values (Region −1st 0th 1st 0 315 15 180 −15 0 270 –30 180 30 0 225 −75 180 75 0 180 −120 180 120 180 315 −165 0 165 180 270 150 0 −150 180 225 105 0 −105 180 180 60 0 −60
The far-field scattering pattern of the space-time digital metasurface at the th order harmonic can be written as [47]
From the phase distributions listed in Table 1, the far-field scattering patterns under normal incidence are calculated by Eq. (6) for the different harmonics, as shown in Fig. 4(b). In Fig. 4(b), two symmetric beams at the fundamental frequency, and the vortex beams with hollow intensity at the st order harmonics are generated. To show more details of the harmonic wavefronts, the near-field distributions at the observation plane (located at 1200 mm in front of the metasurface) are calculated and shown in Figs. 4(c) and 4(d). Typical intensity profiles with a hollow center, and spiral phase profiles with different rotation directions can be clearly recognized at the st order harmonics. Besides this, the characteristics of dual beams at the fundamental frequency can also be observed. The calculated profiles for both far and near fields again demonstrate the great potential of the metasurface for nonlinear wavefront controls.
3. EXPERIMENT AND DISCUSSION
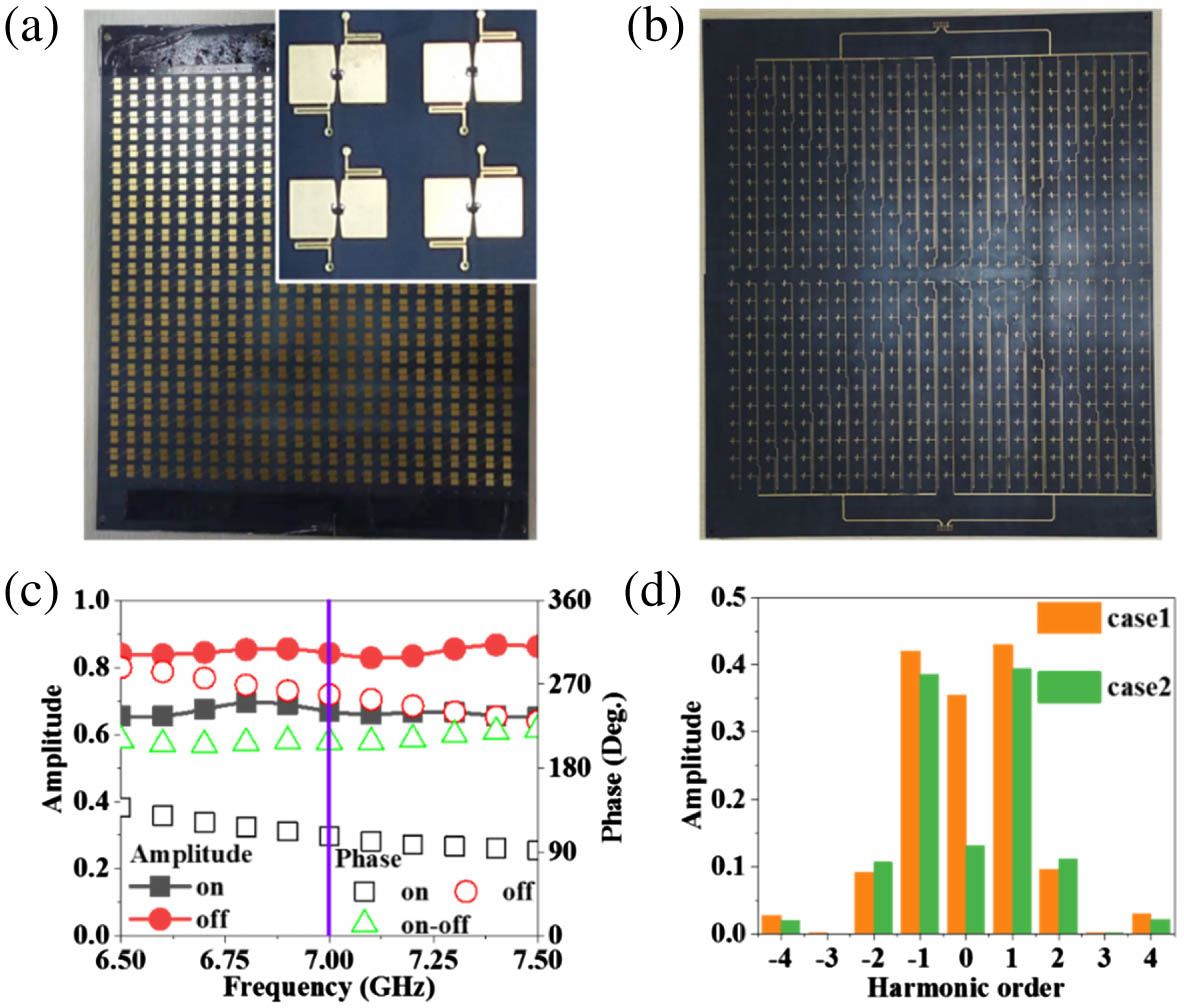
Fig. 5. (a) Front view and (b) back view of the proposed space-time digital coding metasurface. (c) Measured reflection spectra for the on and off states of the PIN diodes. (d) Measured harmonic amplitude distributions under two coding sequences 111100000000 and 000011111111, respectively.
In the first experiment, all the metaatoms are turned on or off simultaneously to measure the reflection amplitudes and phases of 0 and 1 states, as shown in Fig. 5(c). At 7 GHz, the measured amplitudes and phases of the metasurface are approximately 0.7 and 100°, and 0.84 and 260° for the on and off states of the PIN diodes, respectively. The measured phase difference between the two states is 200°, which is slightly greater than the desired 180° in theory.
In the second experiment, the reflection spectra under the periodic phase modulation are measured, where two standard horn antennas are employed to transmit and receive signals. One antenna is connected to a microwave signal generator (Keysight E8267D), which provides the excitation signal at 7 GHz. Another horn antenna is used to receive the harmonic scattered signals via a spectrum analyzer (Keysight E4447A). The control circuit is programmed to generate square-wave control signals with the desired biasing voltages, periods, and time delays. The modulation period of the coding sequences is 100 μs with the modulation frequency .
Two cases are considered in the experiment, in which the basic coding sequence is 000011111111. The initial phase is selected as 0° for the case 1, and 180° for the case 2, leading to the changed coding sequence 111100000000 in the case 2. The measured amplitude spectra in the two cases are shown in Fig. 5(d). From Eq. (2), the amplitude spectra should not be affected by the change of . In Fig. 5(d), the harmonic amplitudes are nearly equal except at the fundamental frequency. This can be ascribed to phase deviations of the metaatoms from their ideal values under different biasing voltages, stemming primarily from the parasitic parameters of the PIN diode, the fabrication tolerance, and the distortion of the control signal.

Fig. 6. (a) Schematic of the experimental setup for measuring the harmonic near-field distributions. (b) Photograph of the nonlinear near-field experiment environment. (c) The coaxial probe for near-field detection. (d) The waveguide antenna as the excitation of the metasurface. (e), (f) Measured amplitude and phase distributions of the fundamental and st order harmonics.
The experiment configuration of the nonlinear near field mapping system is shown in Fig. 6(b). The excitation antenna is placed at a distance of 700 mm above the metasurface. The coaxial probe is shown in Fig. 6(c). The scanning plane is located at 1200 mm away from the metasurface, with a scan range of (scanning step: 20 mm). The eight regions of the metasurface can be independently controlled by the FPGA control board. The initial coding sequence was 000011111111 with the modulation frequency , which corresponds to the combination of values and . When and , the coding sequence is changed to 111100000000.
Figures 6(e) and 6(f) illustrate the measured harmonic magnitude/phase distributions of the electric fields where and are listed in Table 1. As expected by the theoretical analysis, the typical intensity profiles with a hollow center, and the spiral phase profiles with different rotation directions can be clearly observed in Figs. 6(e) and 6(f), indicating the generation of OAM beams with the topological number for st order harmonics. The measured distributions at fundamental frequency are different from the calculated results, which can be ascribed to the huge gap of intensity between the case 1 and case 2 in Fig. 5(d).
There are two main factors responsible for the deviation between the calculated and measured results. (1) The calculated near-field patterns in Figs. 4(c) and 4(d) are obtained under the incidence of plane wave, and the harmonic phases along the metasurface can be accurately determined. However, in the experiment, the feeding source is a waveguide antenna, and it is not a strictly plane wave when arriving at the metasurface. Therefore, the harmonic phase profile is disturbed which may cause the opposite influence on the generations of OAM beams at different harmonics. (2) The measurement of harmonic amplitudes and phases is a great challenge in our experiment. First, the harmonic amplitudes are minimal, and they cannot be directly measured by the VNA. The amplitude/phase comparison method is adopted to solve this problem where a comparing probe is introduced near the detection plane. The coaxial probe with omnidirectional pattern has low efficiency to obtain the harmonic energies. Besides, the slight vibration of the probe during the movement can also affect the measurement accuracy. (3) Other factors, including parasitic parameters of the PIN diodes, the distortions of the control signals, the fabrication tolerances can also lead to the deviations of the harmonic intensities and phase distributions under the time-varying reflectivity. Nevertheless, from the measured and simulated results, we can still conclude that the time-domain metasurface can manipulate the wavefronts at the fundamental and high-order harmonics independently.
4. CONCLUSIONS
In this paper, we propose a space-time digital metasurface to realize near-field manipulations of the fundamental and st order harmonics. Under the normal incidence of a plane wave, the metasurface can generate two symmetric beams at fundamental frequency, and two OAM beams with the topological number at the st order harmonics simultaneously. A novel experimental platform for nonlinear field mapping is developed to measure the harmonic near fields of the space-time digital metasurface. Although our experiments are conducted at microwave frequencies, the approach can be further extended into the THz and visible regimes by incorporating advanced modulation technologies.
Jin Yang, Jun Chen Ke, Mao Chen, Ming Zheng Chen, Jun Yan Dai, Jian Feng Chen, Rui Yang, Jun Wei Wu, Qiang Cheng, Tie Jun Cui. Control of the harmonic near-field distributions by an active metasurface loaded with pin diodes[J]. Photonics Research, 2021, 9(3): 03000344.