Detection and upconversion of three-dimensional MMW/THz images to the visible
Download: 1374次
1. INTRODUCTION
The use of millimeter wave (MMW) and terahertz (THz) radiation has increased in recent years, especially in the fields of spectroscopy [1] and imaging [2,3]. More and more applications in medicine, communications, homeland security, material science, and space technology are based on MMW and THz radiation bands [24" target="_self" style="display: inline;">–
There are already several existing room-temperature MMW and THz detectors [2,3]. The most popular detectors are Golay cells, pyroelectric detectors, bolometers, and microbolometers, many of which are too slow for video frame rates. Among those detectors, the vanadium oxide (VO
The GDD’s detection mechanism is based on a slight change of current between the two electrodes of the lamp; these current changes occur due to the electric field of the electromagnetic radiation being incident on the GDD. The expression for the change in bias current of the GDD due to MMW/THz radiation is given by Ref. [12]: where
A new (to our knowledge) principle of detection and imaging using the miniature GDD is presented here in which we detect the change in the light emission from the GDD due to incident MMW/THz radiation rather than the change in its electrical current. By using the GDD for the conversion of MMW/THz radiation to the visual band, it is shown that the response time of the detection can be improved, since it is now mainly dependent on the visual detector response time rather than the GDD circuit. An advantage of this method is the elimination of the internal shot noise of the GDD components [12], which limits its sensitivity when measuring the change in its electrical current. Thus, the sensitivity and minimum detectable signal power using the upconverting (optical) method will improve the GDD sensitivity and simplify the detection signal compared to electronic detection. A method for microwave power distribution detection was published this year [17], using a one-dimensional array of arc discharge lamps that serves as an energy converter from microwave to visible light. The high-power microwave radiation can produce visible light in the lamps. The range of illumination in the lamps over the lamp array depends on the microwave intensity. Thus, the microwave distribution can be monitored by optical observation. Our work is in the MMW/THz band and shows the ability of our method to use GDDs as pixels in an imaging system. Another MMW imaging technique based on visible light emitted from the positive column of a Cs–Xe DC discharge was shown in Ref. [18]. In that technique, MMW radiation is incident toward a large, uniform positive column window and causes changes in the positive column light intensity. A charge-coupled device (CCD) camera is used to obtain the MMW pattern. The minimum detectable MMW power density of this system was found to be on the order of
This paper presents the ability of our MMW/THz imaging system to obtain 3D images using upconversion. This ability was experimentally tested by performing detection, imaging, and frequency modulation continuous wave (FMCW) radar detection using a GDD and a photodetector.
2. EXPERIMENTAL SETUP AND RESULTS
2.1 A. Detection and Proof of Concept
In order to investigate the upconversion of MMW/THz radiation to visual light using GDD phenomena, we used a MMW/THz source based on multipliers from Virginia Diodes, Inc., that multiply a low-frequency source to 100 GHz. The 100 GHz source radiates to free space and, using a polyethylene (PE) lens, the MMW/THz radiation was focused on the GDD cross section between the electrodes. The experimental setup is given in Fig.
The detected optical intensity signal from the photodetector output for the upconversion detection using the N523 GDD is shown in Fig.
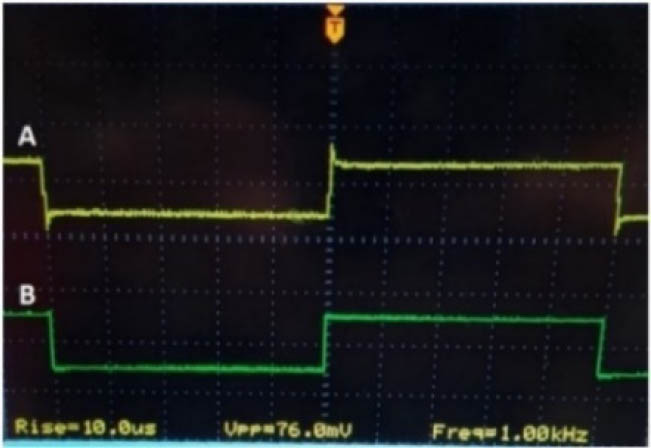
Fig. 2. Detected signal from the photodetector (signal A, 76 mV peak to peak) and modulation signal of the MMW/THz radiation (signal B) on the same time axis.
In order to find the best GDD lamp for the upconversion method, we tested many GDD lamps from different vendors. Several of them contained a phosphorous coating on the inner surface of the glass envelope, and several of them were without the phosphorous coating. The N523 was found to be the best one. This lamp has a phosphorous coating. Note that similar results were found for electronic detection [5].
Figure
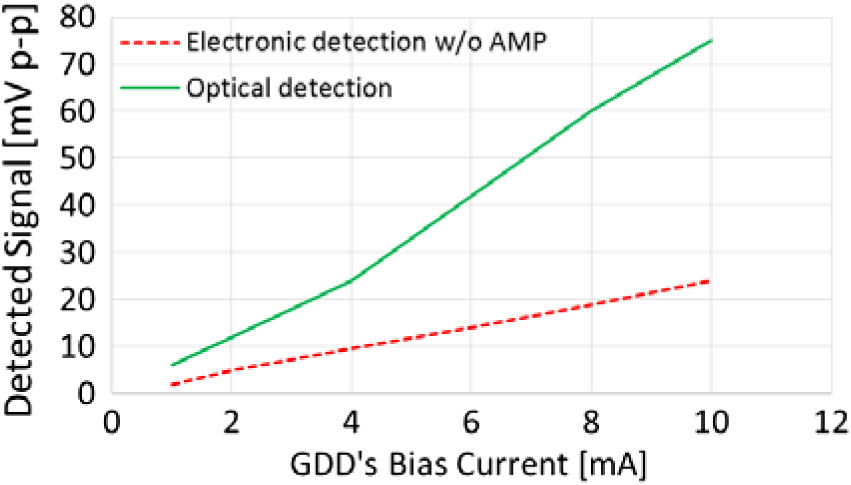
Fig. 3. Detected signal from the photodetector (solid line) and the detected signal from the electronic circuit without amplifier (dashed line) as a function of the GDD DC bias current.
The responsivity of the system was calculated by measuring the input MMW/THz power on the GDD cross section, as well as the output signal voltage from the photodetector. In order to measure and calculate the input MMW/THz power, we used an MMW/THz pyroelectric array camera from Spiricon to measure the beam diameter. The beam power was measured using an MMW/THz absolute power meter from Thomas Keating. The total MMW/THz radiation power incident on this cross section was calculated to be 800 μW. The detected signal from the photodetector output was measured to be 76 mV, which results in a responsivity of 95 V/W for the detection system at 100 GHz with a GDD bias current of 10 mA; for comparison, the detected signal from the electronic circuit (without an amplifier) was measured to be 25 mV, which results in a responsivity of 31 V/W for the electronic detection system at 100 GHz with the same GDD bias current. The optical upconversion was thus more responsive than the electronic detection.
The optical polarization sensitivity of the GDD using the upconversion method was investigated in side configuration [7]. The maximum detection value was obtained when the DC electric field was in the direction of the MMW/THz electric field. The minimum detection value was obtained when the fields were orthogonal to each other. The minimum detection value was 30% of the maximum detection value. This optical polarization sensitivity is similar to the electronic polarization sensitivity, as shown in previous works [7,15,20,21], and is in good agreement with Malus’s law. When MMW/THz frequencies are such that wavelength is on the order of electrode geometry dimensions, absorption by the plasma and noticeable responsivity increases can occur [21].
A calculation of the system noise-equivalent power (NEP) was carried out using
The noise levels of the detection system were measured with a spectrum analyzer from Agilent Technologies. The noise voltage was measured to be about
Figure
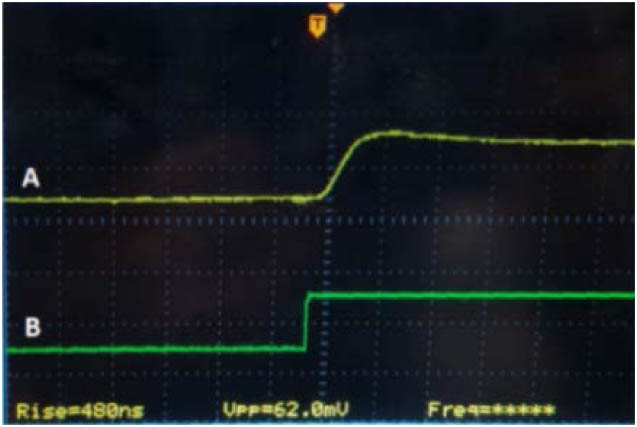
Fig. 4. Detected signal from the photodetector (signal A) and modulation signal of the MMW/THz radiation (signal B). The response time of the detection using the PDB210A photodetector was found to be 480 ns.
2.2 B. Imaging Using Upconversion
Figures
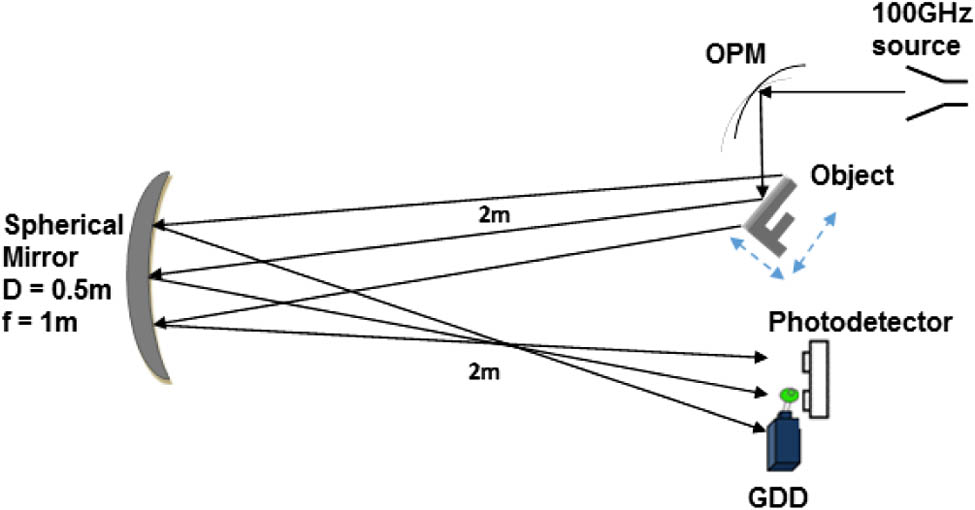
Fig. 5. Setup configuration for the upconversion imaging system using a GDD and photodetector.
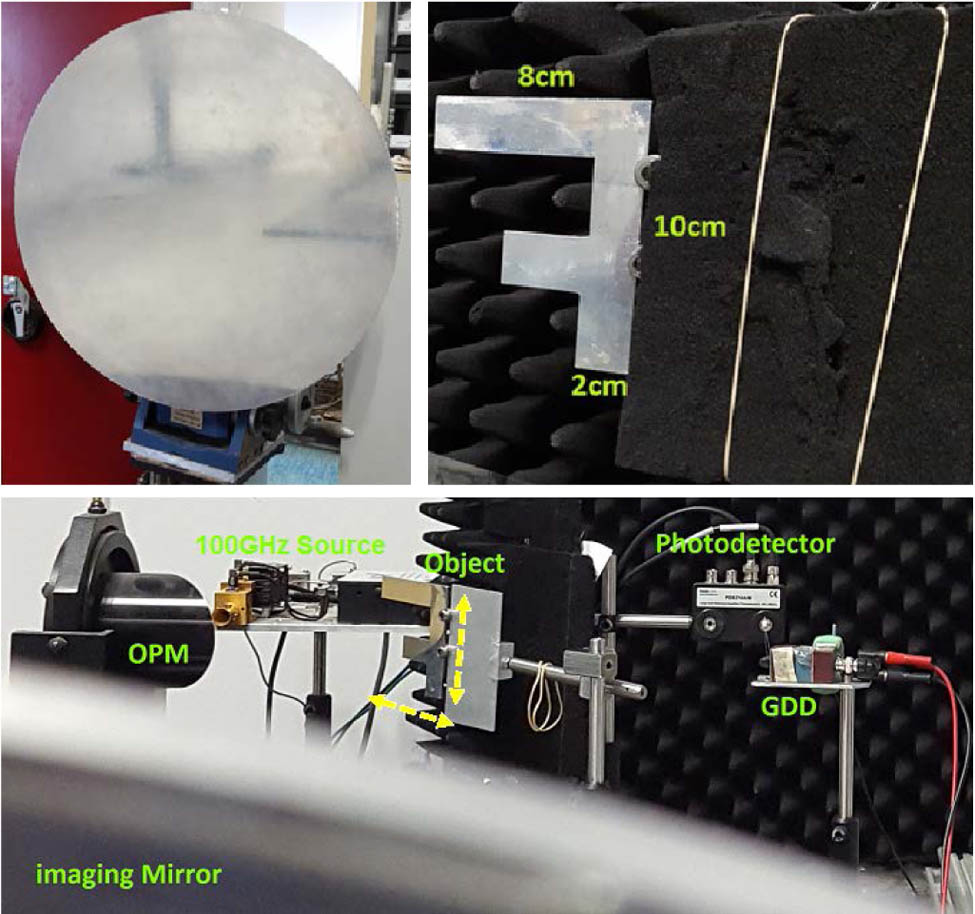
Fig. 6. Pictures of the setup configuration for the upconversion imaging system using a GDD and photodetector, imaging mirror, and metal object with a size of and letter width of 2 cm.
Figure

Fig. 7. Imaging results: (a) the raw upconverted MMW/THz image, (b) the image after thresholding low values.
2.3 C. FMCW Detection Using Upconversion
FMCW radar systems employ frequency modulation at the signal source to enable propagation delay measurements for determination of the distance to the target or depth of an object in an imaging system. In order to realize FMCW radar, the signal source radiation is split into two beams. The first beam is incident toward the object and reflected back to the detector and is called the signal beam. The second beam is incident directly on the detector and is called the reference beam. Either the signal beam or the reference beam is frequency modulated (chirped), so that the time difference in propagation (
According to the beat frequency (
The depth resolution, designated as
Figure
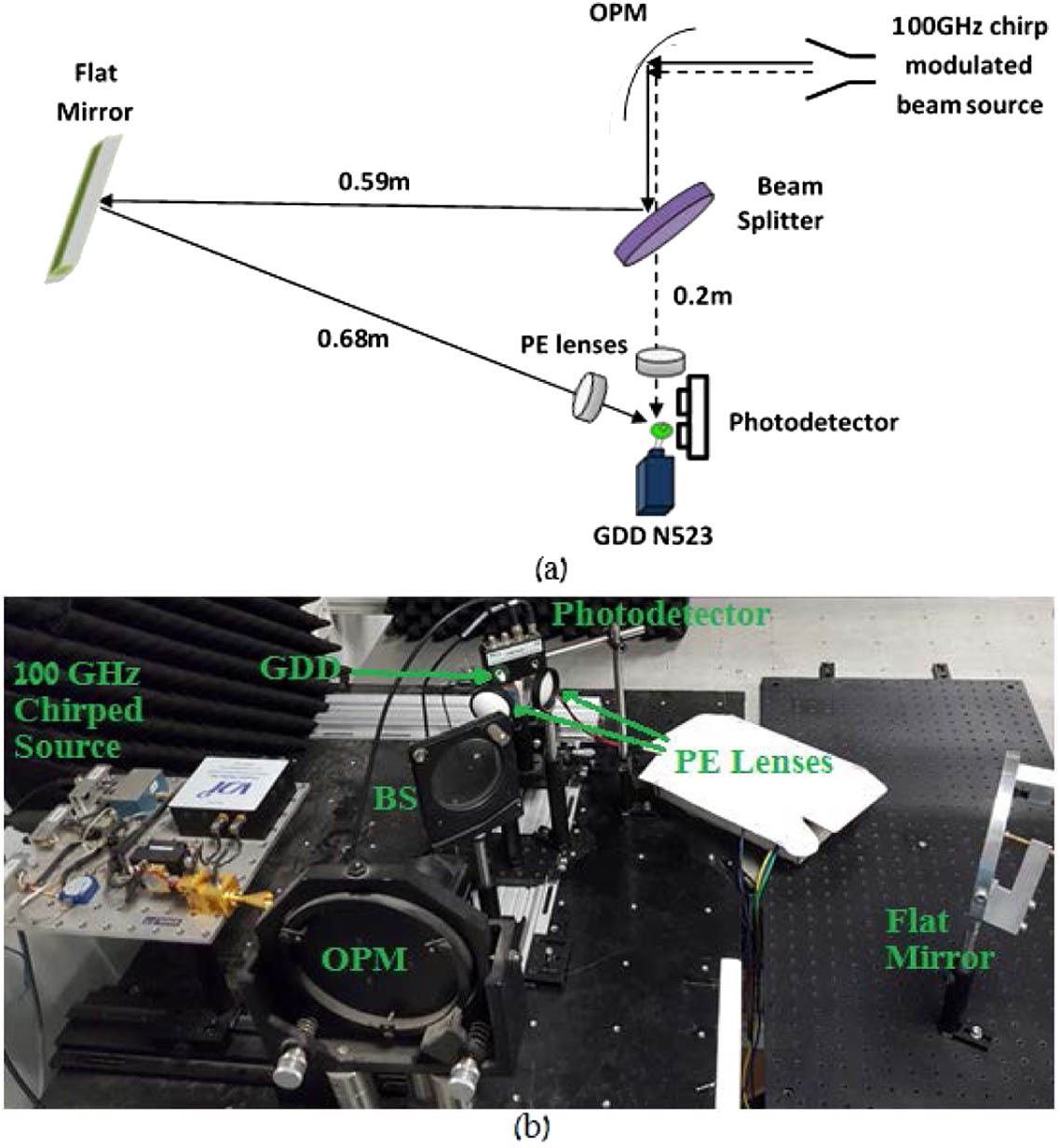
Fig. 8. Setup configuration for an optical FMCW experiment at 100 GHz using a photodetector and GDD lamp N523 in side configuration, connected to the detection electronic circuit and external amplifier.
In this experiment, according to our measurement conditions, the chirp bandwidth was about
In this experiment we measured the detected signal from the photodetector and that from the electronic circuit (with external amplifier) and compared them. Figure
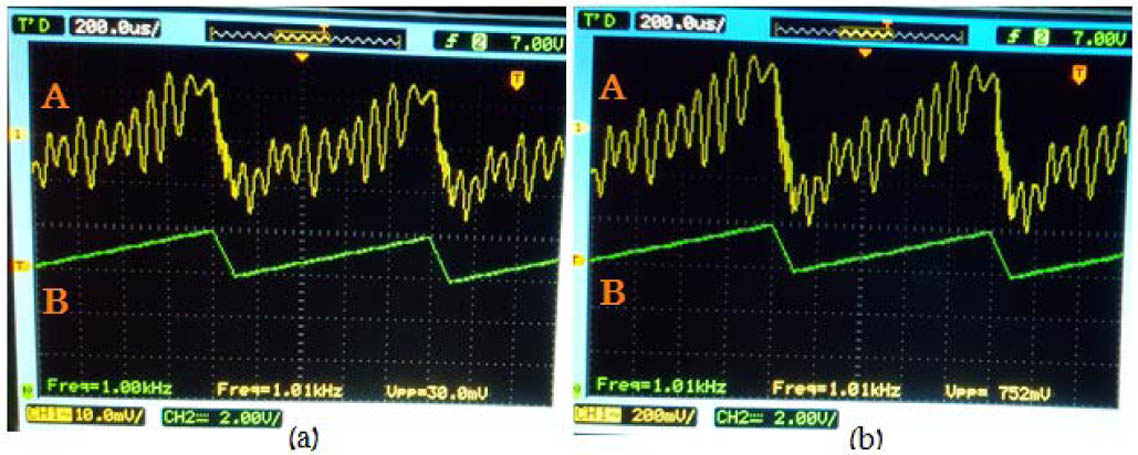
Fig. 9. Detected signal A and modulation signal B for the FMCW experiment: (a) upconversion optical heterodyne detection, (b) electronic heterodyne detection.
The raw data of the FMCW signal recorded by the oscilloscope was analyzed using a fast Fourier transform (FFT), which shows the frequency components of the detected signal. Figure
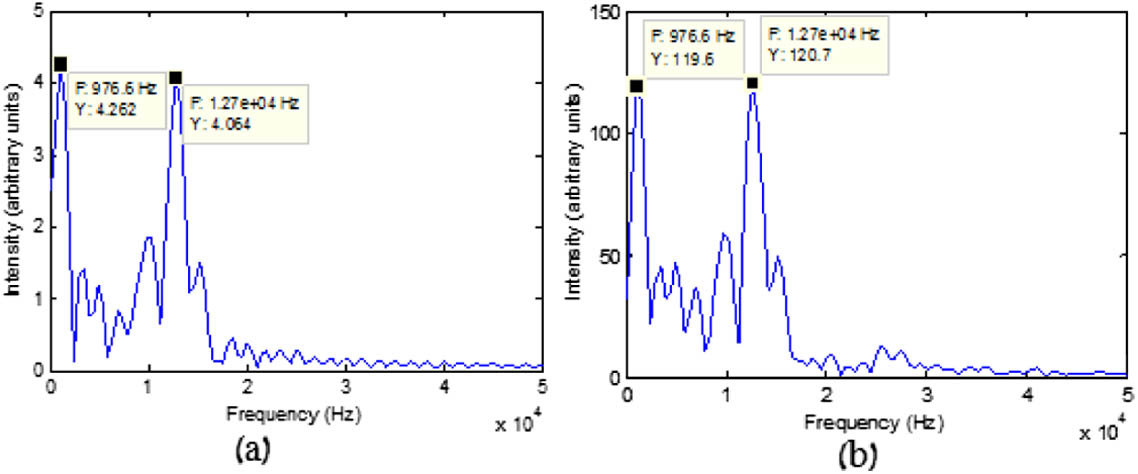
Fig. 10. FFT of the detected signal from the FMCW experiment: (a) upconversion optical heterodyne detection, (b) electronic heterodyne detection.
3. DISCUSSION AND CONCLUSIONS
The experimental results of this paper show that a system with a GDD and optical detector can serve for MMW/THz imaging. The results show improvement of the response time using the presented method compared to the electronic detection of the GDD. Furthermore, the NEP using this method was almost an order of magnitude lower than the NEP in the electronic detection, and the responsivity was 3 times better than in the electronic detection. The photodetector is much quieter than the high bias GDD. It was found that the upconversion method using the GDD is sensitive to the polarization of the incident MMW/THz radiation, similar to the electronic detection. The response time results that were shown in our work were limited due to the bandwidth of the optical detector, which affects the performance of the detection. Improvement of the upconversion system can be achieved by using better optical components and reducing the size of the optical system (to bring the optical detector closer to the GDD); further improvement of the response time and sensitivity can be achieved using a faster and more sensitive optical detector. Also, testing other GDD models using this method can result in finding better Ne miniature lamps for detection by this method rather than electronic detection.
One important application of the GDD is as a pixel in a MMW/THz FPA [25]. In this work we showed and proved the ability of this method to perform imaging using a GDD lamp and photodetector in a scanning system. We believe that based on the work presented in this paper we can use a CCD camera and a GDD FPA to perform imaging. The combination of a CCD camera and a GDD FPA can yield a faster, more sensitive, and very inexpensive MMW and THz camera, eliminating the complexity of the electronic circuits and the internal electronic noise of the GDD. By choosing lamps with smaller diameters, we can achieve better spatial resolution with an FPA. Comparison of our system to another imaging system using an upconversion method described before in Ref. [18] shows that the sensitivity of our imaging technique exceeds the other technique’s sensitivity by about 6 orders of magnitude (by comparing the minimum detectable signal power densities of both systems). Also, our system response time is about 2 times better than that of the other technique. This makes our upconversion system much better for MMW/THz imaging. A major difference between the large lamp in Ref. [18] and the miniature GDD lamps used here is the lamp dimensions. In our present case, the very small electrode separation (
Furthermore, we succeeded in performing optical FMCW detection using a photodetector. The detected signal that was measured was consistent with theory. From the comparison of both detection methods we can see that the optical detection performed well and agreed with the electronic detection beat frequency. Using heterodyne and advanced detection methods, we can achieve better results with optical detection. The optically detected signal in this experiment can be enhanced by better positioning of the photodetector. These results indicate that upconversion of an MMW/THz image to the visible range using glow discharge miniature neon indicator lamps can be enhanced by FMCW techniques to obtain fast and sensitive 3D images.
In electronic detection, the input to the GDD is an electromagnetic wave, and the output is an electrical current. Therefore, limitations in response time in electronic detection derive from parasitic capacitance and inductance of the GDD. With upconversion, the output from the GDD is an optical wave rather than a current, so parasitic impedance is decoupled from the output, and the optical detection speed derives largely from the speed of the optical detector. Similarly, electronic detection sensitivity is largely limited by GDD plasma noise. With upconversion, this too is decoupled, and sensitivity is largely limited by optical detector noise, which is much less than plasma noise. In both cases, we are measuring detection properties of the upconversion system rather than those of the GDD device alone. Thus, both speed and sensitivity can be improved further by use of a detector or optical camera that is quieter and faster.
[3] F. Sizov. THz radiation sensors. Opto-Electron. Rev., 2009, 18: 10-36.
[4] P. H. Siegel. Terahertz technology. IEEE Trans. Microwave Theory Tech., 2002, 50: 910-928.
[12] N. S. Kopeika. Glow discharge detection of long wavelength electromagnetic radiation: cascade ionization process internal signal gain and temporal and spectral response properties. IEEE Trans. Plasma Sci., 1978, PS-6: 139-157.
[13] A. Abramovich, N. S. Kopeika, D. Rozban, E. Farber. Terahertz detection mechanism of in expensive sensitive glow discharge detector. Appl. Phys., 2008, 103: 093306.
[19] N. S. Kopeika, N. H. Farhat. Video detection of millimeter waves with glow discharge tubes: part I—physical description; part II—experimental results. IEEE Trans. Electron. Devices, 1975, ED-22: 534-548.
[20] A. Aharon (Akram), D. Rozban, N. Banay, A. Abramovich, N. S. Kopeika, A. Levanon. Polarization effects on heterodyne detection and imaging using glow discharge detector at millimeter wavelengths. Proc. SPIE, 2014, 9078: 90780F.
[23]
[24]
Article Outline
Avihai Aharon (Akram), Daniel Rozban, Avi Klein, Amir Abramovich, Yitzhak Yitzhaky, Natan S. Kopeika. Detection and upconversion of three-dimensional MMW/THz images to the visible[J]. Photonics Research, 2016, 4(6): 06000306.