Regular uniform large-area subwavelength nanogratings fabricated by the interference of two femtosecond laser beams via cylindrical lens
Download: 715次
Laser-induced periodic surface structures have been extensively studied in various materials[1
Laser interference lithography has been widely applied to the fabrication of large-area micro/nanogratings[19,20]. To fabricate a grating with interference spacing less than the laser wavelength, the angle between two laser beams must be larger than 60°. For a femtosecond laser, the focus diameter is usually only tens of microns (μm), and this requires that the straightness of three translation stages should be less than 10 μm in total for excellent interference fringes. However, it is not economical to achieve this extremely high precision in the industry. In this study, we built a double cylindrical lens interference setup with a small angle of 9.3° based on an 800 nm, 50 fs, 3.5 mJ, and 1 kHz laser system (Legend Elite, Coherent). The interference spacing is 4.94 μm, seven times the wavelength of a surface plasmon polariton (SPP). Interestingly, regular uniform nanogratings with a period of SPP wavelength, instead of the interference period, were fabricated at the scanning velocity of . In addition, the graphic pattern of “raining of petals” and two types of flowers with nanogratings in different directions are fabricated, where the silicon wafer is mounted on an four-axis translation stage. These flowers exhibit bright pure colors observed with a charge-coupled device (CCD) in different directions.
Figure
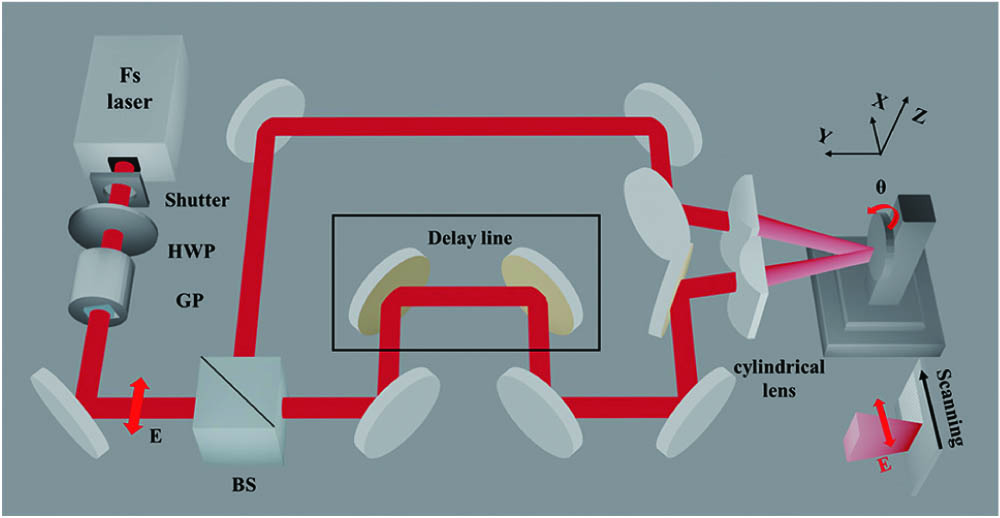
Fig. 1. The experimental setup of femtosecond laser interference via two cylindrical lenses. HWP is half-wave plate, GP is Glan prism, and BS is beam splitter. The double arrow E represents laser polarization.
The sample used in this study is a commercially available undoped Si wafer (100) of diameter 100 mm and thickness 0.5 mm. The surface is optically polished with a roughness of . Before laser processing, the silicon wafer was dipped in an acetone solution and sonicated for 5 min to remove the contaminating impurities and was then washed with pure water. After laser irradiation, the surface nanostructures were rinsed in hydrofluoric (HF) acid solution (5 mol/L) for 30 min to remove the oxidation and the ablation fragments before measuring with scanning electron microscopy (SEM)[17,21].
After single pulse radiation, only interference stripes of size 50 μm are found in the ablation area. These interference stripes cause SPP excitation during the second laser pulse radiation and induce shallow subwavelength ripples that cover the interference stripes themselves. After three laser pulse radiations, very regularly spaced nanogratings of period are formed on the interference stripes, as shown in Fig.

Fig. 2. Microstructures in the ablation area after radiation of three laser pulses. (b)–(e) Enlarged SEM pictures of the areas in squares in (a). The double arrow in (e) represents laser polarization. Laser fluence of single beam is , and the scale bars are 5 μm.
The SPP excitation causes periodic distribution of the light field and further induces subwavelength periodic ripples; in addition, the SPP wavelength greatly varies with the dielectric constant in the excited state[2]. Because of the Gaussian distribution of the light field, the local dielectric constant, as well as the ripple period, at the center is much different from that at the edge of the ablation spot[16]. However, the SEM images in Figs.
During laser irradiation, a large amount of debris and surface defects are generated on the ablation spot, which greatly affects the SPP propagation, resulting in curved ripples with many breaks and bifurcations[18,19]. However, the nanogratings are very straight and continuous, despite the presence of some deposited particles. The ripple periods are mostly similar at about , which do not change greatly with local laser fluence. Moreover, the nanogratings are very straight and continuous, despite the presence of deposited particles. These phenomena are very interesting, but also much unexpected.
Subwavelength periodic ripples are effectively generated on the surface of silicon by direct writing with a single laser beam. Figure
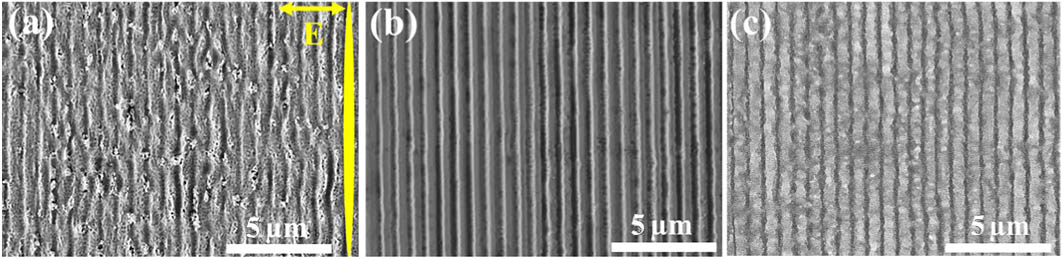
Fig. 3. (a) Subwavelength periodic ripples by direct writing with a single laser beam. (b) RUSNGs ripples fabricated by direct writing of two-beam interference. (c) RUSNGs ripples without etching in HF solution. The double arrow E represents laser polarization, and the narrow ellipse represents the laser focus.

Fig. 4. Different types of micro/nanostructures depending on the laser fluence and scanning velocity.
Interestingly, the periods of all RUSNGs are similar (), irrespective of any higher value of laser fluence and scanning velocity, as presented in Fig.
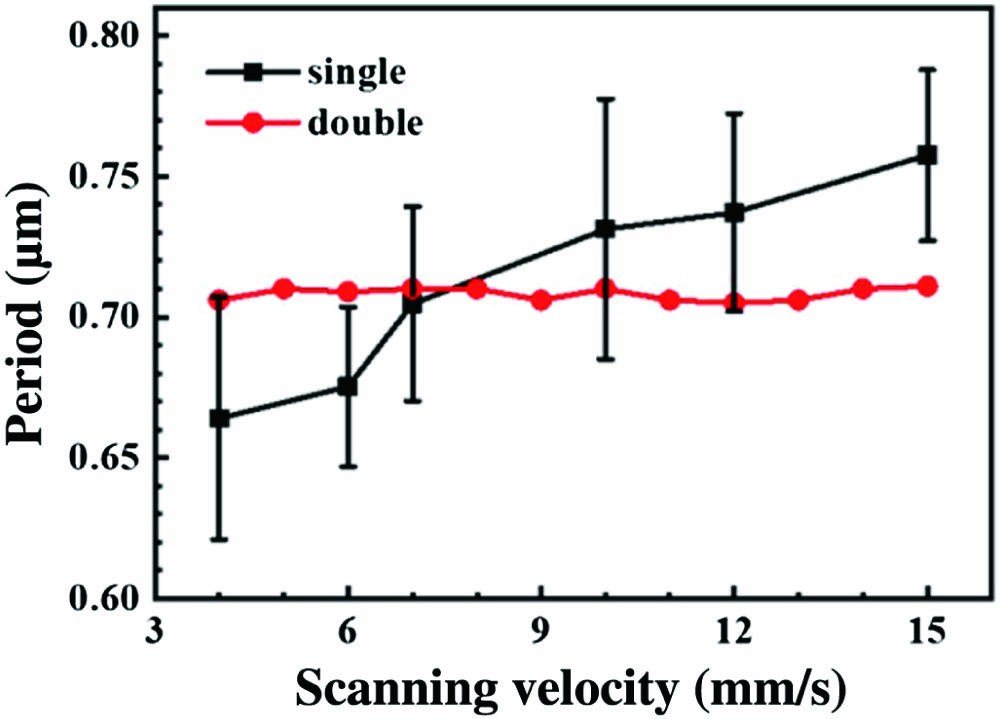
Fig. 5. Periods of subwavelength ripples as a function of scanning velocity induced by a single laser beam and two-beam interference.
The interference period is 4.94 μm, seven times the SPP wavelengths. When the size of a microcavity is an integer multiple of surface plasmon wavelengths, the local field intensity enhances greatly for the resonance enhancement. We propose that the resonance-enhanced SPP imposes a strong constraint on the generation of periodic ripples and has an excellent anti-disturbance ability. The formation mechanism of RUSNGs should be studied in detail in the future (Supplementary Material 1).
The RUSNGs in a large area are fabricated by direct writing of two-beam interference by scanning the sample in a succession of lines[22,23]. Figure

Fig. 6. (a) Optical image of the colored surface and (b) SEM image of RUSNGs. The scale bar is 5 μm.
To test the performance of the RUSNGs fabricated by this method, a wide-spectrum light source (, tungsten halogen lamp) is perpendicularly illuminated on the sample surface, and the diffraction spectra are measured using a fiber spectrometer at different angles, as shown in Fig.

Fig. 7. Optical characterization measurements of the periodic ripples. (I) Diagrammatic sketch of the diffraction spectra test; diffraction spectra of (a) subwavelength ripples generated by single laser beam and (b) RUSNGs.
Two different samples, subwavelength ripples generated by scanning a single laser beam, and the RUSNGs fabricated by two-beam interference, are mounted at the same stage in sequence. The measured diffraction spectra are shown in Fig.
The Commission Internationale de l’Eclairage (CIE) chromaticity diagram is presented in Fig.
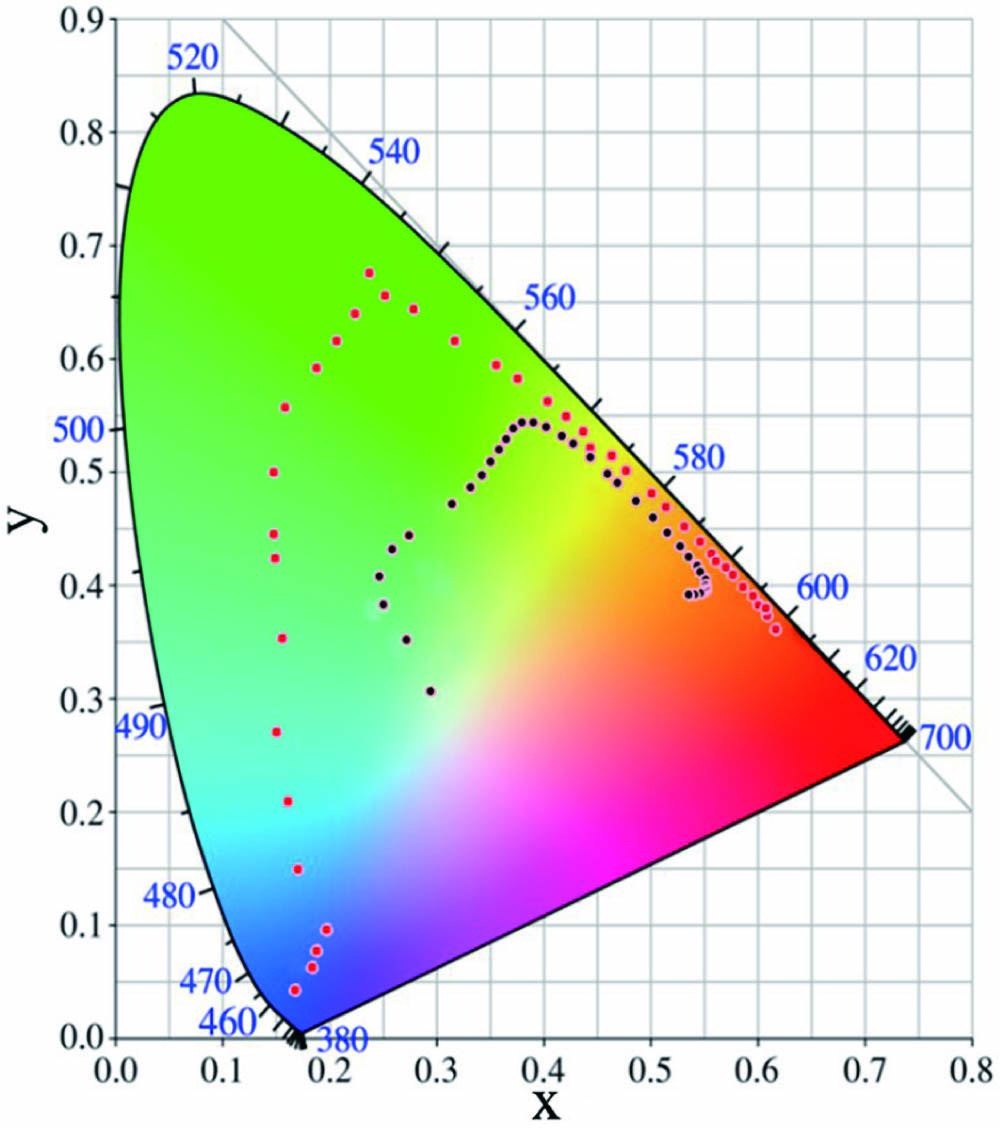
Fig. 8. CIE chromaticity diagram showing the colors of the RUSNGs (red dots) and subwavelength ripples (black dots).
Different patterns in tin foil are fabricated by two-dimensional (2D) laser scanning and cover on the Si wafer. The RUSNGs are formed in patterns by direct writing of two-beam interference, which presents more excellent structural colors[6,25]. Figure
By adjusting the azimuth angle of the laser focus relative to the center of the rotatory stage and scanning the sample in the horizontal direction, nanogratings in different directions can be fabricated. A schematic of the processing method is shown in Fig.
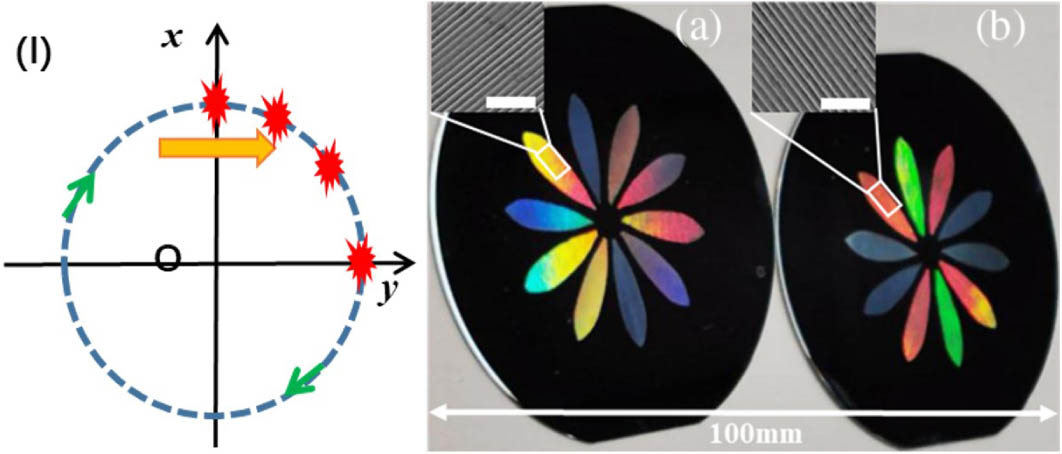
Fig. 10. Colorful optical images of two flowers with nanogratings in different directions. (I) Schematic of the processing method. Flowers with nanogratings in (a) azimuth direction and (b) radial direction. The two enlarged SEM pictures represent the nanogratings in the corresponding squares. The scale bars are 5 μm.
In conclusion, this study demonstrated an efficient method to fabricate RUSNGs by two-beam interference via two cylindrical lenses. The interference period was adjusted to be an integer multiple of the SPP wavelength, and RUSNGs with a period of were fabricated on a silicon wafer with the scanning velocity of . Complex cross-scale patterns with RUSNGs in different directions were fabricated when the wafer was mounted on an four-axis translation stage. These patterns exhibited vivid structural colors at different angles. The processing method proposed in this study presents potential applications in the fields of surface structural colors, birefringent optical elements, data storage, broadband absorption, and enhanced photoluminescence.
[1]
[2]
[3]
[4]
[5]
[6]
[7]
[8]
[9]
[10]
[11]
[12]
[13]
[14]
[15]
[16]
[17]
[18]
[19]
[20]
[21]
[22]
[23]
[24]
[25]
Kaiqiang Cao, Long Chen, Ke Cheng, Zhenrong Sun, Tianqing Jia. Regular uniform large-area subwavelength nanogratings fabricated by the interference of two femtosecond laser beams via cylindrical lens[J]. Chinese Optics Letters, 2020, 18(9): 093201.