Superconductivity in La and Y hydrides: Remaining questions to experiment and theory
1 I. INTRODUCTION
The attainment of room-temperature superconductivity is a long-standing challenge related historically to the metallic phase of hydrogen.
After the BCS mechanism was established,
However, attempts to find superconducting hydrides have produced another idea involving the concept of “doped” metallic hydrogen, which is usually attributed to Gilman and Ashcroft.
The highest Tc values have been predicted so far for compounds of La and Y: LaH10 and YH10,
2 II. OVERVIEW OF THEORETICAL AND EXPERIMENTAL DATA FOR LaH10
As with all superhydrides measured so far, theoretical predictions come first. For example, the theoretical predictions of Tc in clathrate-like structured hydrides were published as early as 2012 for CaH6.
![Clathrate structures of typical metal superhydrides (polyhydrides).21 Maximum Tc is predicted for YH10 and is slightly above room temperature at 250 GPa. [Reprinted Figs. 2 and 4 with permission from Peng et al., Phys. Rev. Lett. 119(10), 107001 (2017). Copyright (2017) The American Physical Society.]](/NV_LEGCY/images/figure.jpg)
Fig. 1. Clathrate structures of typical metal superhydrides (polyhydrides).21 Maximum Tc is predicted for YH10 and is slightly above room temperature at 250 GPa. [Reprinted Figs. 2 and 4 with permission from Peng et al. , Phys. Rev. Lett. 119 (10), 107001 (2017). Copyright (2017) The American Physical Society.]
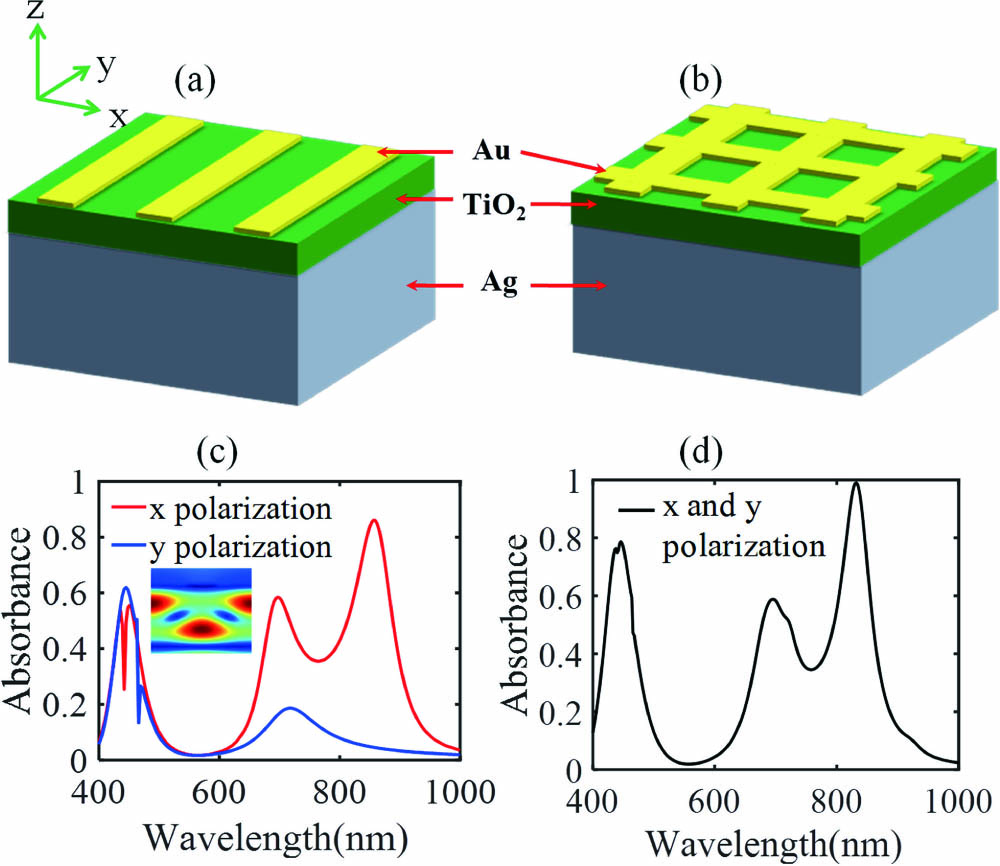
Fig. 2. Predicted superconductivity in LaH10 and YH10 superhydrides.22 Blue and red curves and symbols correspond to the indicated values of the Morel–Anderson pseudopotential, μ*.
![X-ray diffraction of LaH10 sample after laser heating at 175 GPa and 169 GPa.24 Right panel: Volume per formula unit compared with theory and sum of the volumes of pure La and five H2 units. [Reproduced with permission from Geballe et al., Angew. Chem., Int. Ed. 57, 688–692 (2018). Copyright 2018 Wiley-VCH Verlag GmbH & Co. KGaA.]](/NV_LEGCY/images/figure.jpg)
Fig. 3. X-ray diffraction of LaH10 sample after laser heating at 175 GPa and 169 GPa.24 Right panel: Volume per formula unit compared with theory and sum of the volumes of pure La and five H2 units. [Reproduced with permission from Geballe et al. , Angew. Chem., Int. Ed. 57 , 688–692 (2018). Copyright 2018 Wiley-VCH Verlag GmbH & Co. KGaA.]
The experimental confirmation of an Fm-3mLaH10 structure quickly followed in the publication by Geballe et al.
The first reports of superconductivity in LaH10 appeared as arXiv publications. Drozdov et al. submitted a brief report of a resistivity drop at ∼215 K in laser-heated samples loaded in a hydrogen pressure medium.
Drozdov et al. published another arXiv paper, claiming LaH10 with a maximum Tc of about 254 K,
The experimental setting for resistivity measurements using the four-probe technique from Somayazulu et al.
![Resistivity studies of superconductivity in LaH10.25 Left panel: Typical arrangement of electrical contacts and x-ray transmission scan through the sample and contact area. Right panel: Resistivity signatures of superconductivity in LaH10. [Reprinted Figs. S1 and 2 with permission from Somayazulu et al., Phys. Rev. Lett. 122(2), 027001 (2019). Copyright 2019 The American Physical Society.]](/NV_LEGCY/images/figure.jpg)
Fig. 4. Resistivity studies of superconductivity in LaH10.25 Left panel: Typical arrangement of electrical contacts and x-ray transmission scan through the sample and contact area. Right panel: Resistivity signatures of superconductivity in LaH10. [Reprinted Figs. S1 and 2 with permission from Somayazulu et al. , Phys. Rev. Lett. 122 (2), 027001 (2019). Copyright 2019 The American Physical Society.]
![Observed volumes per formula unit before and after the conversion from La to LaH10±x for several samples probed by resistivity technique.25 The predicted P–V curves for the assemblages La + 5H2 and La + 4H2 are plotted as the dashed and dash-dot lines (respectively). Samples which do not show evidence of the conductivity drop above 80 K lie below the La + 4H2 line. Right panel shows the resistivity of one of the samples under varying pressure during thermal cycling (supplementary material in Ref. 25). [Reprinted Figs. S3 and S4 with permission from Somayazulu et al., Phys. Rev. Lett. 122(2), 027001 (2019). Copyright 2019 The American Physical Society.]](/NV_LEGCY/images/figure.jpg)
Fig. 5. Observed volumes per formula unit before and after the conversion from La to LaH10±x for several samples probed by resistivity technique.25 The predicted P–V curves for the assemblages La + 5H2 and La + 4H2 are plotted as the dashed and dash-dot lines (respectively). Samples which do not show evidence of the conductivity drop above 80 K lie below the La + 4H2 line. Right panel shows the resistivity of one of the samples under varying pressure during thermal cycling (supplementary material in Ref. 25 ). [Reprinted Figs. S3 and S4 with permission from Somayazulu et al. , Phys. Rev. Lett. 122 (2), 027001 (2019). Copyright 2019 The American Physical Society.]
Drozdov et al.
For the LaH10 phase, Drozdov et al.
![Transition to a lower symmetry phase (R-3m) in LaH10 on decompression from ∼170 GPa.24 [Reproduced with permission from Geballe et al., Angew. Chem., Int. Ed. 57, 688–692 (2018). Copyright 2018 Wiley-VCH Verlag GmbH & Co. KGaA.]](/NV_LEGCY/images/figure.jpg)
Fig. 6. Transition to a lower symmetry phase (R-3m) in LaH10 on decompression from ∼170 GPa.24 [Reproduced with permission from Geballe et al ., Angew. Chem., Int. Ed. 57 , 688–692 (2018). Copyright 2018 Wiley-VCH Verlag GmbH & Co. KGaA.]
Magnetic susceptibility measurements have not been attempted for LaH10 due to the very small volume of the synthesized samples—see Ref.
3 III. MAGNETIC SUSCEPTIBILITY STUDIES AT HIGH PRESSURE IN LaH10
The technique we use for magnetic susceptibility measurements is a double-modulation technique introduced originally by Timofeev,
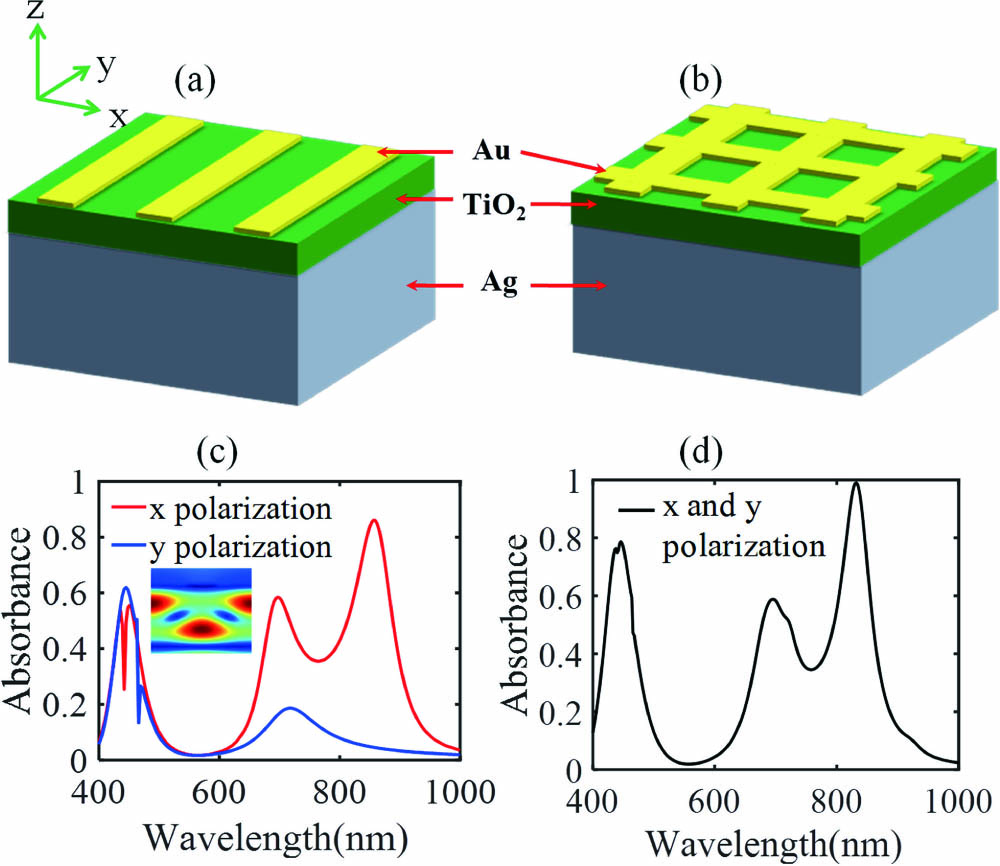
Fig. 7. Left panel: Schematic representation of the background subtraction principle in magnetic susceptibility measurements: 1. primary coil; 2. secondary compensating coil; 3. secondary signal coil. Further experimental details can be found in Ref. 38 . “Removal” of the sample from the signal coil by applying an external magnetic field over the critical value produces measurable changes in the total output signal. On the right we show a typical signal of a cuprate superconductor compared with a model calculation for type II superconductors—see Ref. 38 for details of the model calculation.
We were able to detect a measurable signal, however, the estimated sample size proved to be very small. We show raw data at several pressures in
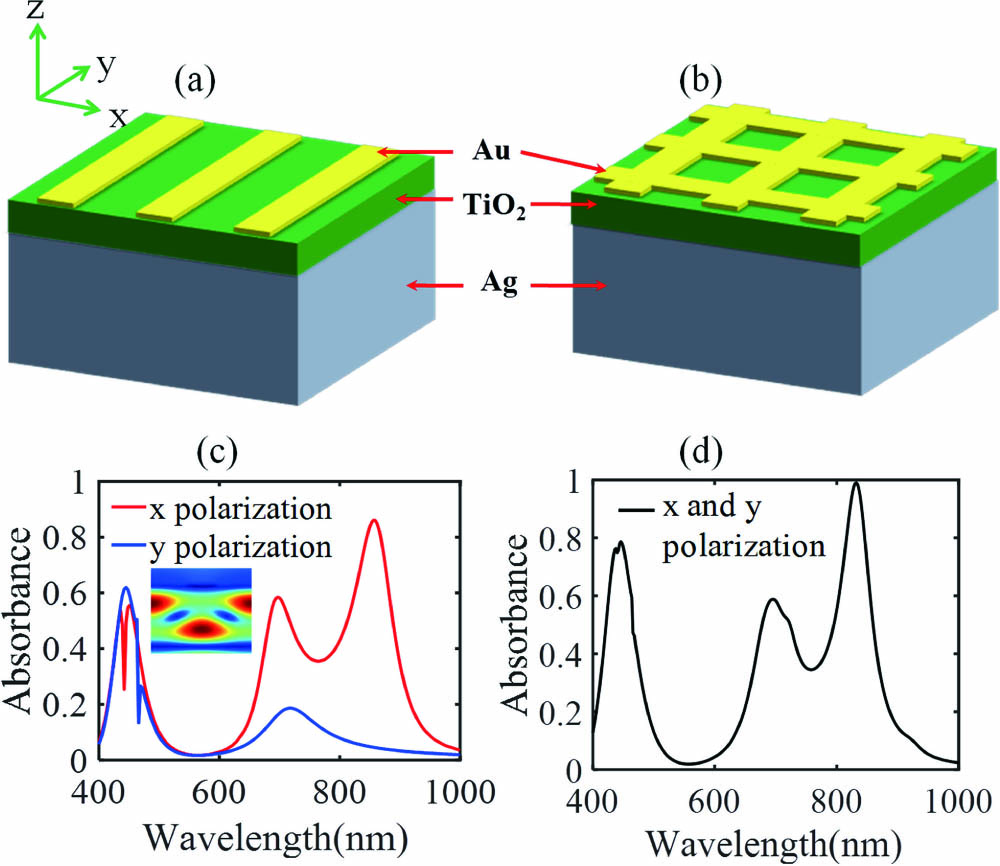
Fig. 8. Left panel shows the magnetic response signals from the LaH10 sample in a DAC. The superimposed signals at several pressures are shown by dots: blue—164 GPa, green—169 GPa, red—180 GPa; the background is shown as a red dashed line (approximated by 3rd degree polynomial). Right panel: Signal after background subtraction, shifted in vertical direction (from bottom to top: blue—164 GPa, green—169 GPa, red—180 GPa).
We have estimated sample size using previous measurements from larger samples of high-Tc superconductors and MgB2 samples (
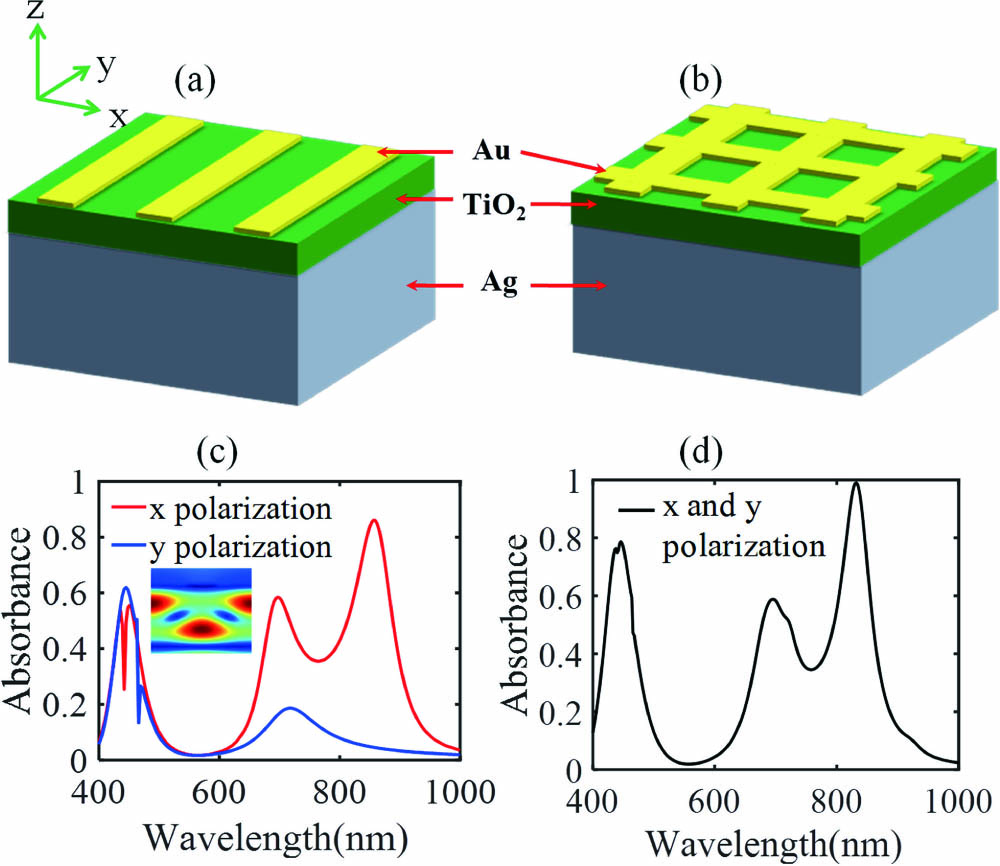
Fig. 9. Calibration curves for two setups operating at two different excitation frequencies.35 The samples used for calibrating the amplitude of the response are various high-Tc cuprates, Nb, and MgB2. The practical sensitivity limit for the 160 KHz setup is around 10−10 – 2 × 10−10 cm3, which is more than one order of magnitude lower in comparison with the typical sensitivity limit (10−8 e.m.u.) of an MPMS system from Quantum Design.
We summarize the available experimental data for LaH10 samples in
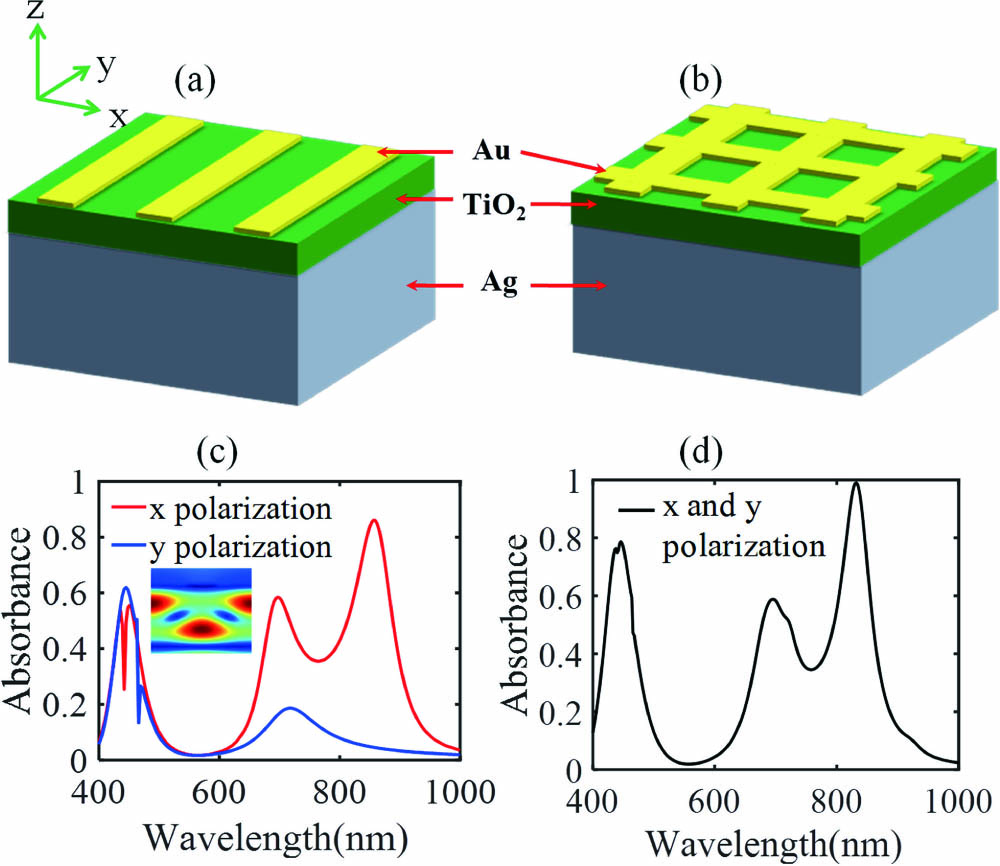
Fig. 10. Summary of the available experimental and theoretical data of superconductivity in LaH10. Sources for the data: Somayazulu et al. ,25 Drozdov I et al. ,32 Drozdov II et al. ,26 Liu et al. ,22 Kruglov et al. ,40 and Peng et al .21
Indeed, the existence of a phase transition in the 160–170 GPa pressure range, which is driven by a soft mode (dynamical instability), may explain an increase in Tc in the relatively narrow pressure range above the transition. An indirect confirmation can be invoked from the theoretical calculations of Tc in LaH10 by Kruglov et al.
In the recent arXiv contribution, Errea et al.
Kruglov et al.
4 IV. NEW SUPERCONDUCTING SUPERHYDRIDES YH6 AND ThH10
In a recent arXiv publication, Troyan et al.
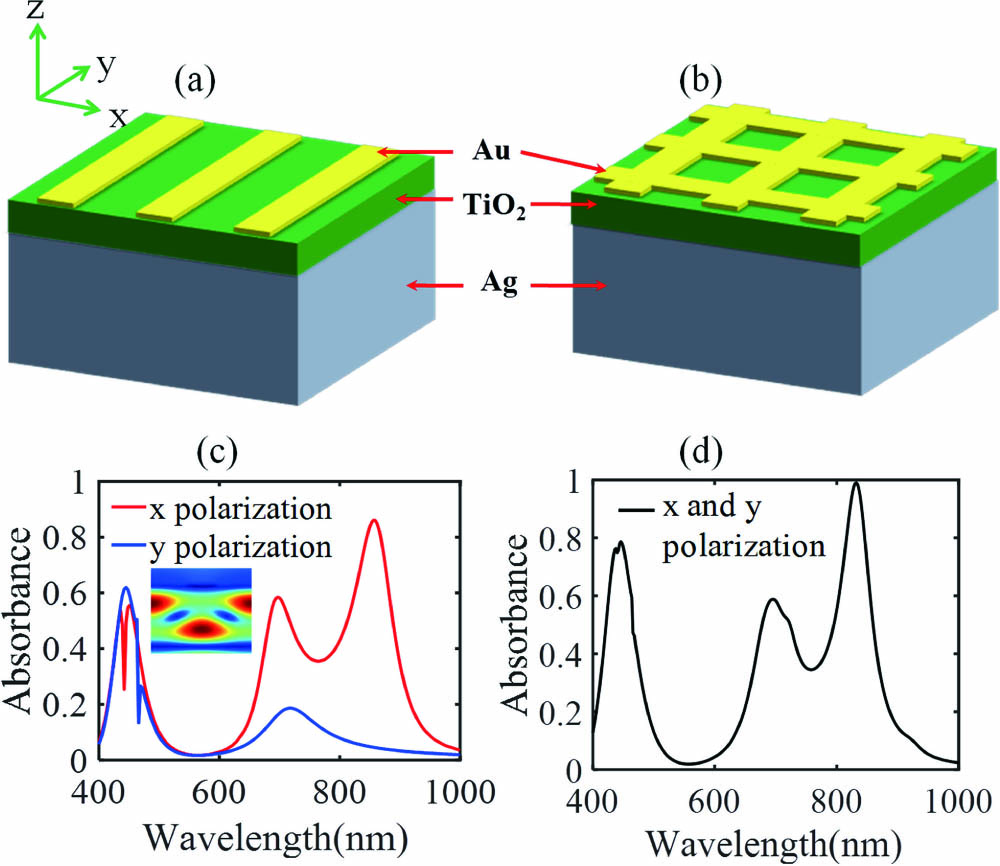
Fig. 11. Troyan et al. 41 (a) XRD pattern of M3 sample at 172 GPa recorded at λ = 0.2952 Å; (b) Le Bail refinements for Imm -YH6 and I 4/mmm -YH4. Red circles are experimental data; black line is the fit; green line shows residues.
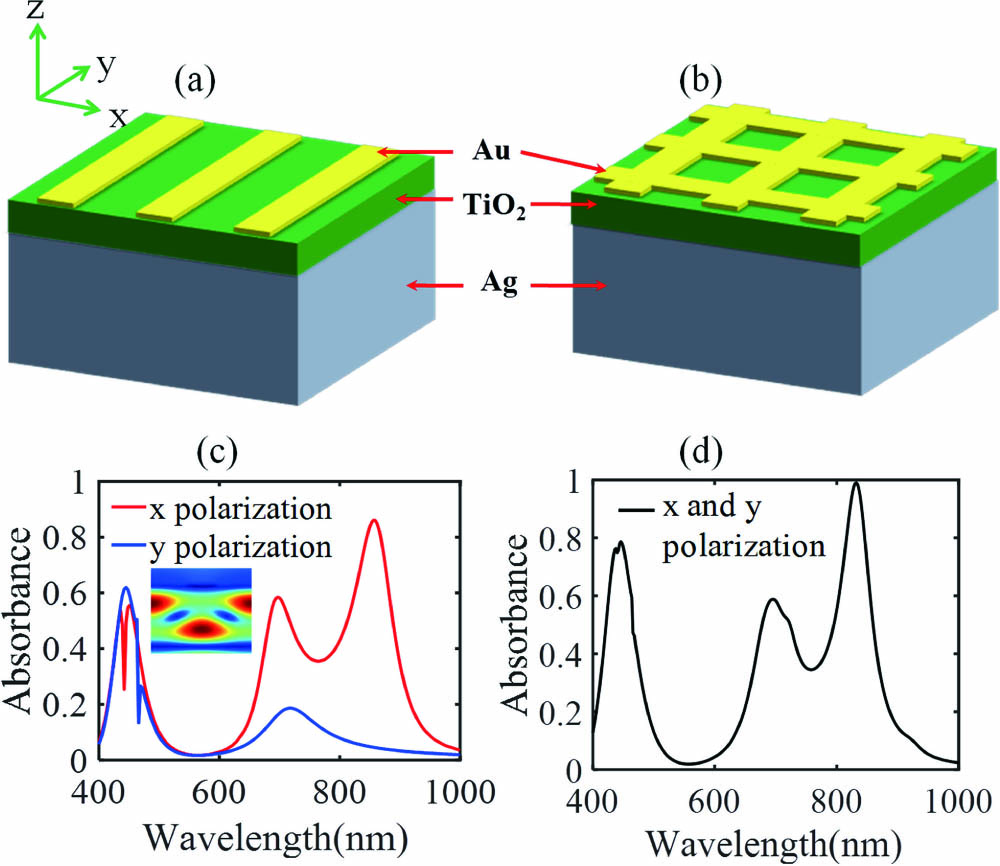
Fig. 12. Troyan et al. 41 Superconducting transitions in Imm -YH6: (a) Dependence of electrical resistance on temperature. Inset: the resistance drops to zero after cooling below ТC ; (b) jump in R (T ) dependence of resistance (nine times increase) on temperature for the second sample.
Another superconducting hydrides report was released in arXiv in 2019 by Semenok et al.
![Semenok et al.44 Observation of superconductivity in (a) ThH10 and (b) ThH9. The temperature dependence of the resistance (R) of thorium superhydride was determined in a sample synthesized from Th+NH3BH3. The resistance was measured using four electrodes deposited on a diamond anvil with the sample placed on top of the electrodes [Fig. 15(a), inset] with an excitation current of 100 µA. The resistance near the zero point is shown on a smaller scale in the insets; (c) dependence of the resistance on temperature under an external magnetic field at 170 GPa; (d) dependence of the critical temperature (Tc) of ThH10 and ThH9 on the magnetic field.](/NV_LEGCY/images/figure.jpg)
Fig. 13. Semenok et al. 44 Observation of superconductivity in (a) ThH10 and (b) ThH9. The temperature dependence of the resistance (R) of thorium superhydride was determined in a sample synthesized from Th+NH3BH3. The resistance was measured using four electrodes deposited on a diamond anvil with the sample placed on top of the electrodes [Fig. 15(a) , inset] with an excitation current of 100 µ A. The resistance near the zero point is shown on a smaller scale in the insets; (c) dependence of the resistance on temperature under an external magnetic field at 170 GPa; (d) dependence of the critical temperature (Tc) of ThH10 and ThH9 on the magnetic field.
5 V. PERSPECTIVES FOR FUTURE ROOM-TEMPERATURE SUPERCONDUCTIVITY STUDIES
With the available experimental data on superconducting superhydrides, we may summarize a few empirical facts related to their structural and superconducting properties. In
![High-Tc superconducting superhydrides,47 which is very similar to the highest Tc in elements under high pressure conditions (see e.g., http://www.hpr.stec.es.osaka-u.ac.jp/e-super) [The figure is reprinted with permission from supplementary material in Semenok et al., J. Phys. Chem. Lett. 9(8), 1920–1926 (2018). Copyright 2018 American Chemical Society.].](/NV_LEGCY/images/figure.jpg)
Fig. 14. High-Tc superconducting superhydrides,47 which is very similar to the highest Tc in elements under high pressure conditions (see e.g., http://www.hpr.stec.es.osaka-u.ac.jp/e-super ) [The figure is reprinted with permission from supplementary material in Semenok et al. , J. Phys. Chem. Lett. 9 (8), 1920–1926 (2018). Copyright 2018 American Chemical Society.].
Another important observation is the systematic trends in the partial volume occupied by the hydrogen atom in high-Tc superhydrides. For example, in
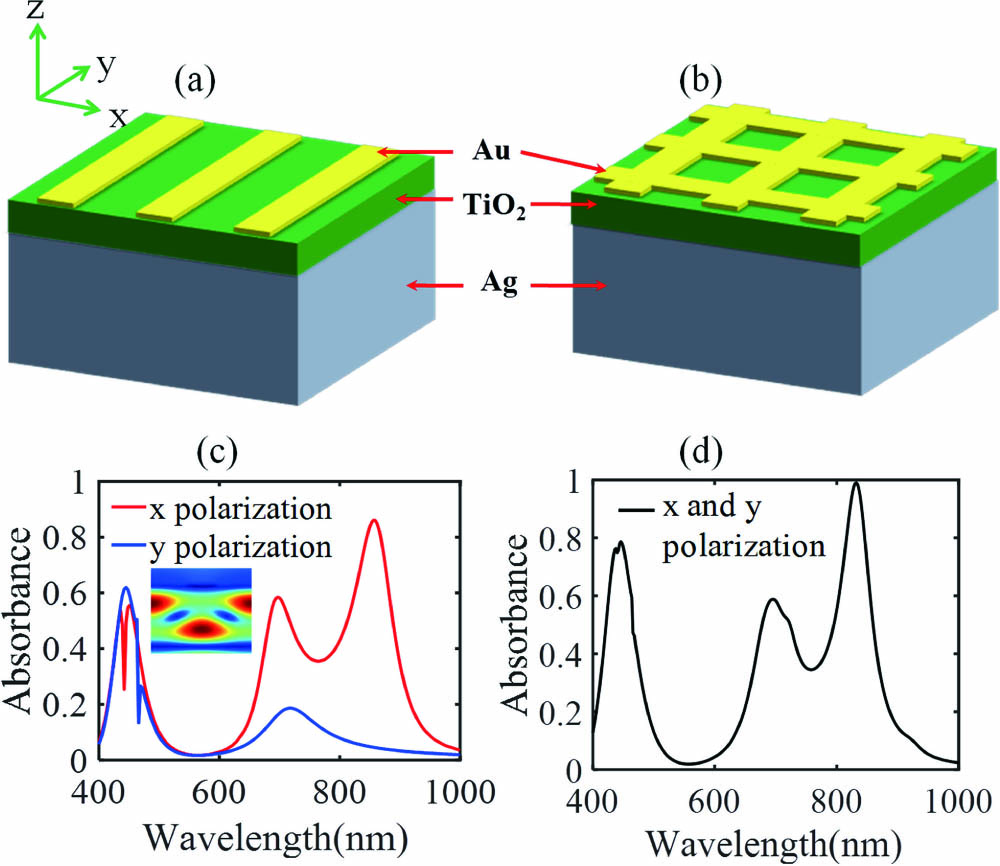
Fig. 15. Comparison of atomic volumes per hydrogen atom in high-Tc and nonsuperconducting hydrides. The equation of state for hypothetical atomic hydrogen (green dashed line) is taken from Pepin et al. ,49 as well as atomic H volumes for FeH3 and FeH5. The H2 volume is from Loubeyre et al. 50 LaH10 and AlH3 data are taken from Geballe et al. 24 ThH10 is taken from Semenok et al. ,44 YH6 from Troyan et al. 41 H3S data are given as calculated in Ref. 51 .
We believe that the volume differences given in
The clathrate hydrogen cages in superhydrides are stable only above 100–150 GPa in most studied materials. In that respect, ThH10 stands out as a predicted viable candidate for high-Tc superconductivity below 100 GPa.
6 VI. CONCLUSION
In this brief review we summarized what is known about new superconducting hydrides (superhydrides) that have exceptionally high critical superconducting temperatures. We provided the latest magnetic susceptibility data for LaH10, showing the possibility of high-Tc phases with Tc’s of up to 280 K. The mechanism for the enhancement of Tc in LaH10 was also suggested, based on a soft mode scenario in the vicinity of the Fm-3m to R-3m phase transition in LaH10.
We introduced new experimental data on YH6 and ThH10 in the context of “doped” metallic hydrogen. It appears that the partial hydrogen volume in such superhydrides may be a good indicator of “doping.” In superhydrides, based on partial volume arguments, we suggest that the hydrogen sublattice may be “overdoped” with respect to (hypothetical) pure metallic hydrogen. Overall, the concept of doping may prove very useful in studying ternary superhydrides and is an excellent tool in tuning the doping levels of the hydrogen sublattice.
While at the moment it appears that superconducting superhydrides lack practical importance due to their stability range in the hundreds of GPa, the scientific challenge of the field is enormous and promises exciting times. Room-temperature superconductivity has almost been achieved experimentally, and increasing numbers of theoretical predictions show significantly high Tc’s, even well above the boiling point of water.
[3]
[11]
[12]
[16]
[27] L. Zhang, et al.. Materials discovery at high pressures. Nat. Rev. Mater., 2017, 2(4): 17005.
[31]
[32]
[33]
[34]
[36] Y. A. Timofeev. Detection of superconductivity in high-pressure diamond anvil cell by magnetic susceptibility technique. Prib. Tekh. Eksper., 1992, 5: 186-189.
[39]
[41]
[42] Y. Li, et al.. Pressure-stabilized superconductive yttrium hydrides. Sci. Rep., 2015, 5: 9948.
[46]
[48]
Article Outline
Viktor Struzhkin, Bing Li, Cheng Ji, Xiao-Jia Chen, Vitali Prakapenka, Eran Greenberg, Ivan Troyan, Alexander Gavriliuk, Ho-kwang Mao. Superconductivity in La and Y hydrides: Remaining questions to experiment and theory[J]. Matter and Radiation at Extremes, 2020, 5(2): 028201.