1.5 J high-beam-quality Nd:LuAG ceramic active mirror laser amplifier
Download: 938次
High-energy repetitive nanosecond pulse lasers with a good beam quality are required for many applications[1
In order to achieve high energy at the repetition rate, the choice of amplifier gain medium and its geometry are important. Until now, several solid-state laser materials[10,11] have been widely used, such as Nd:YAG, Yb:YAG, Nd:YVO4, and Nd:YLF, among which Nd:LuAG is considered to be a potential material for high-energy repetitive lasers[11,12], due to its advantages of a moderate emission cross section, a relatively long fluorescence lifetime of 277 μs, as well as outstanding physical and chemical properties.
Lasers using a Nd:LuAG crystal as the gain medium have been increasingly investigated recently. Typically,
In this Letter, a Nd:LuAG ceramic active mirror disk laser amplifier in a MOPA configuration is investigated. In order to obtain a high-energy laser output while maintaining a good beam quality, image relaying systems and stimulated Brillouin scattering phase conjugation mirrors (SBS-PCMs)[16] are used for wavefront correction. A maximum output energy of 1.5 J in a 10 ns pulse duration is obtained at the repetition rate of 10 Hz when the pump energy is 11.5 J, where a maximum pulse energy more than 1 J has been extracted in the Nd:LuAG amplifier, and a far-field beam spot 1.25 times the diffraction limit (DL) has been achieved.
The schematic diagram of the laser is shown in Fig.

Fig. 1. Schematic diagram of the laser. HR: high reflector, PBS: polarized beam splitter, QWP: quarter-wave plate, LDA: laser diode array, SA: serrated aperture.
After passing through two Faraday isolators, the output laser beam is shaped by a serrated aperture and the pulse energy is reduced to 400 mJ. The beam at the serrated aperture is then relay-imaged to the amplifier surface and the beam is expanded from 7 mm to 31 mm with an imaging system. After the first pass of the gain medium, the laser beam is reduced 2 times by the other image-relay system. The relay-imaged laser beam is focused by a focusing lens of 500 mm into an SBS cell filled with Fluorinert liquid (FC-770, 3M Electronics), where a phase conjugate return is generated and reflected. The polarization of the reflected beam is changed from horizontal to vertical by a quarter-wave plate, while after the second pass amplification the beam exits the amplifier through a polarized beam splitter (PBS).
A Nd:LuAG ceramic disk with a diameter of 64 mm and a thickness of 5.5 mm serves as the amplifier gain, which consists of a 50 mm diameter Nd-doped inner region that is surrounded by a 7 mm wide Sm-doped cladding to minimize amplified spontaneous emission (ASE) loss and prevent parasitic oscillations at high gain. The Nd:LuAG ceramic disk is fabricated by the solid-state sintering method. The powder is first vacuum sintered at 1750°C for 5 h under a vacuum of
The front surface of the ceramic gain that the laser enters at a 15° incidence angle is antireflection (AR)-coated for 1064 nm and high reflection (HR)-coated for 808 nm relative to air, while the back surface is HR-coated for 1064 nm and AR-coated for 808 nm relative to water, where an 808 nm laser diode array is used to pump the gain from the back surface, producing a
The transmission of the amplifier after two passes is measured as 89%, corresponding to a loss factor of
To evaluate the stored energy in the gain, the single-pass small signal gain
Figure
For nanosecond-pulse amplification studies, the amplifier is seeded by the pulsed output from the preamplifier, as is shown in Fig.
The one-pass amplified output laser is focused at a point 450 mm inside the 800 mm long SBS-PCM cell where a phase conjugate return is generated. Figure
The near-field pattern (NFP), far-field pattern (FFP), and pulse duration of the double-pass amplified laser pulse with SBS-PCM are shown in Fig.
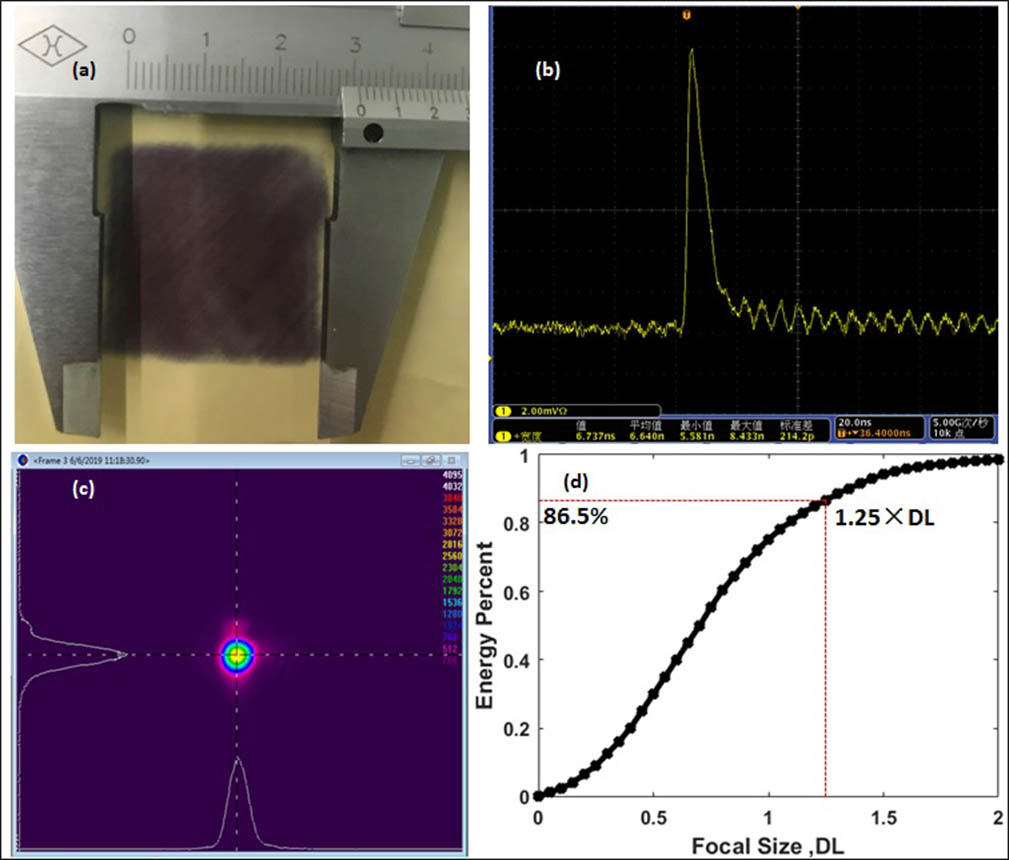
Fig. 6. (a) NFP, (b) compressed pulse duration, (c) FFP, and (d) encircled focal spot energy fraction of the double-pass amplified laser pulse with SBS-PCM.
In summary, we have demonstrated a joule-level Nd:LuAG ceramic active mirror laser amplifier with high beam quality. When the pump energy is 11.5 J, the 1.5 J energy and 6.64 ns pulse at a 10 Hz repetition rate is obtained in double-pass amplification, and the far-field beam spot 1.25 times the DL has been achieved by taking advantage of the SBS-PCM to compensate the laser beam distortion. Our next work will pay more attention to reducing optical scattering losses of the Nd:LuAG ceramic, which will lead to a higher laser output performance.
[1]
[3]
[4]
[5]
[6]
[7]
[8]
[9]
[10]
[11]
[12]
[13]
[14]
[15]
[16]
Jianlei Wang, Kaiqi Zhao, Tao Feng, Xiaolei Zhu, Weibiao Chen. 1.5 J high-beam-quality Nd:LuAG ceramic active mirror laser amplifier[J]. Chinese Optics Letters, 2020, 18(2): 021401.