Subwavelength grating waveguide devices in silicon-on-insulators for integrated microwave photonics (Invited Paper)
Download: 881次
Microwave photonic (MWP) systems exploit the advantages of photonics, especially with regards to ultrabroad bandwidth, adaptability, and parallelism, characteristics that are significantly more challenging to implement in the electronic domain[13" target="_self" style="display: inline;">–
Significant efforts are currently devoted toward developing integrated photonic technologies to realize MWP signal processing functions[3
Recently, there has been growing interest in realizing integrated optical components based on subwavelength grating (SWG) structures[8
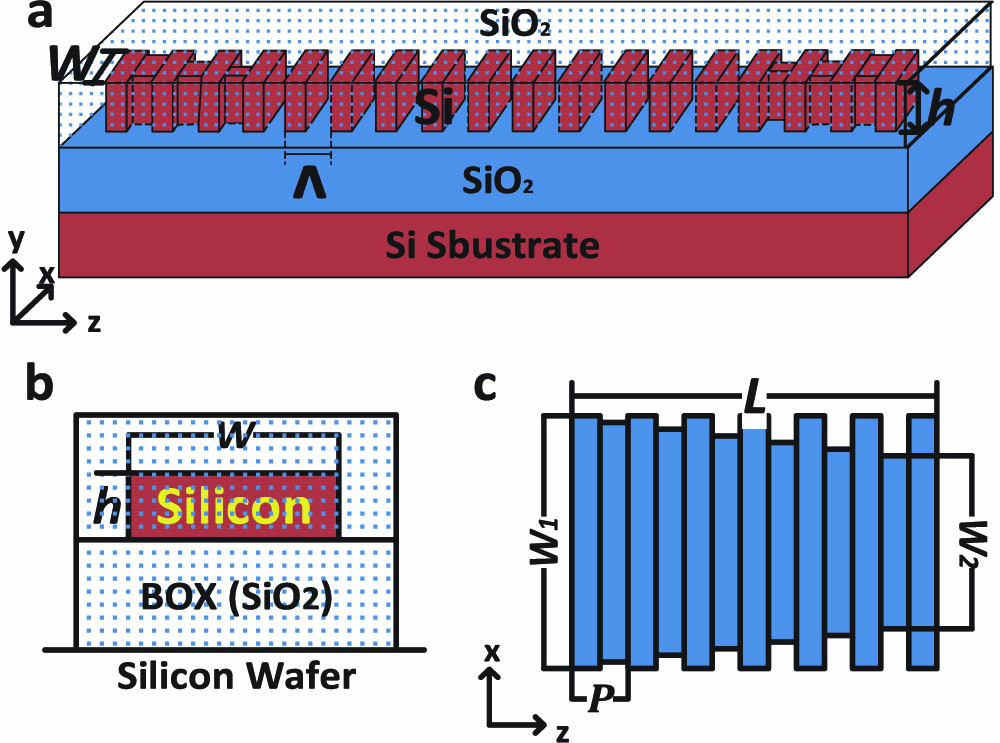
Fig. 1. SWG waveguide in SOI: (a) basic structure, (b) waveguide cross-section, and (c) top view of am SWG taper used to couple light between an SWG waveguide and a conventional waveguide.
To create an SWG waveguide, finite transverse dimensions, e.g., a height
In this paper, we review recent work on realizing basic building blocks based on SWG waveguides in SOI that can be used to implement more complex, integrated MWP signal processing engines. This includes SWG filters such as microring resonators (MRRs), Bragg gratings (BGs), and broadband contradirectional couplers (CDCs), as well as index-variable optical true time delay lines (OTTDLs).
Various MRR-based integrated circuits have been implemented in SOI as well as silicon nitride[5]. Recently, we demonstrated both conventional MRR and racetrack ring resonators based on SWG waveguides in SOI[17].
First, we realized conventional MRRs with add–drop ports based on two SWG bus waveguides and an SWG ring waveguide, as shown in Fig.
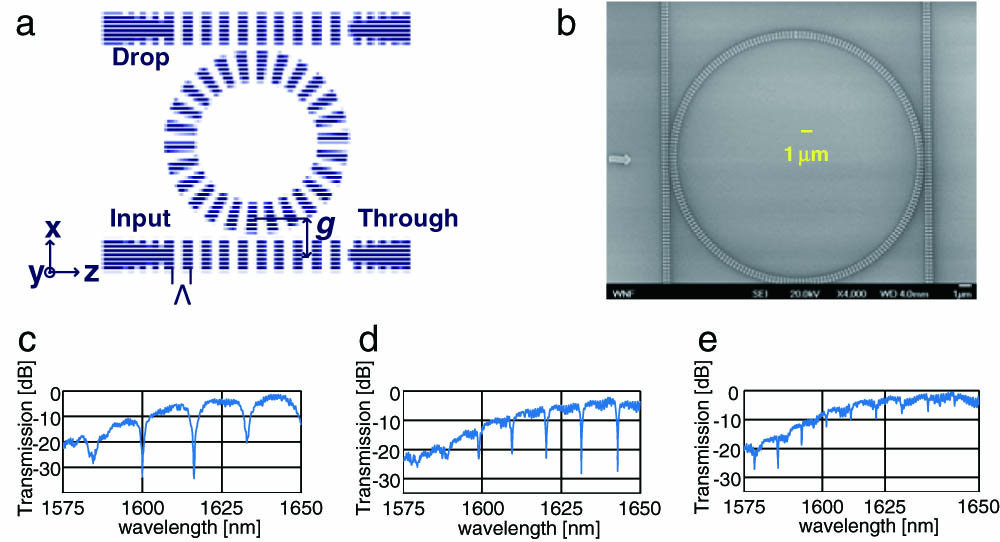
Fig. 2. Conventional MRR based on SWG waveguides in SOI: (a) schematic and (b) SEM prior to top oxide cladding deposition, and measured through responses (normalized) for a ring radius of (c) 15, (d) 20, and (c) 25 μm.
Due to the longer coupling region between the resonator and bus waveguides, racetrack designs may be preferred. Figure

Fig. 3. SWG racetrack resonator: (a) layout, (b) detailed view, and (c) measured through (blue) and drop (red) responses (normalized). See text for device parameters.
For some filtering applications, the periodic nature of MRRs is undesirable. In this context, BGs are more suitable. BGs can be realized by inducing periodic variations in the refractive index along the direction of propagation as well as physical corrugations or variations in the waveguide geometry. We can create a periodic variation in the effective index along the direction of propagation by “interleaving” two SWG waveguides having different duty cycles. Figure

Fig. 4. SWG BG: (a) schematic of SWG BG formed by interleaving two SWG waveguides of different duty cycles, (b) device layout for experimental demonstration, and (c) SEM of the fabricated SWG BG prior to the oxide cladding deposition.
Figure

Fig. 5. (a) Measured transmission spectrum of SWG BG and simple SWG waveguide (see text for parameters). The difference in transmission responses is due in part to the spectral response of the Y-branch (optimized for ), which is present in the SWG BG only. (b) Zoom of the transmission (blue) and reflection (green) responses about the resonant peak at 1546.8 nm.
One drawback of two-port BG devices is that they typically operate in reflection, which usually translates into the need for optical circulators or a Y-branch (splitter/coupler), which, in turn, induces additional optical loss and can impact the gain in an MWP system. On the other hand, wideband BG-defined filtering requirements can be obtained using compact SOI grating-assisted CDCs, which are intrinsically four-port (add–drop) devices[18]. Grating-assisted CDCs are based on two closely spaced (and asymmetric) waveguides and some form of periodic refractive index perturbation along the waveguides. We demonstrate an alternate approach whereby the asymmetric waveguides and grating mechanism are replaced by an SWG waveguide in proximity to a continuous waveguide. In SOI, because of the large optical phase mismatch between the SWG and nanowire waveguides, undesired codirectional coupling can be efficiently suppressed. Moreover, the SWG waveguide provides the required grating mechanism and enables contradirectional coupling. The strong index variation in the SWG leads to a relatively strong coupling coefficient such that power transfers can be obtained using relatively short coupling lengths.
The schematic of the proposed design and the layout of a fabricated device are illustrated in Figs.
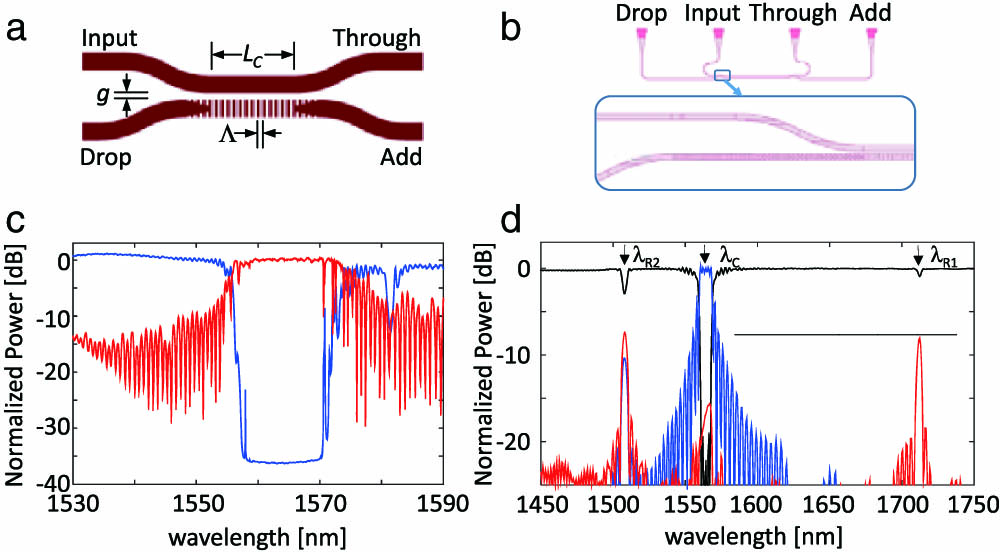
Fig. 6. (a) Schematic of the proposed SWG CDC in SOI, (b) layout of a device with , (c) measured drop (red) and through (blue) responses (see text for device parameters), and (d) 3D FDTD simulated response for SWG CDC with , , and .
The MRRs, BGs, and CDCs are fundamental building blocks that have immediate application in MWP signal processing as well as in implementing more complex devices and systems. For example, due to their wavelength-selective nature, the amplitude response of MRRs and BGs can implement frequency discrimination filters for phase modulation-to-intensity modulation conversion with applications for UWB waveform generation[2]. MRRs can be cascaded to obtain higher-order filter responses or to provide time delays[5]. A serial array of BGs at different resonant wavelengths can also be used to implement a discretely tunable delay line[19]. Cascaded MRRs with different resonant wavelengths (i.e., unique wavelengths within an FSR) or BGs can be used as spectral shapers for generating chirped microwave waveforms based on the principle of spectral shaping followed by wavelength-to-time mapping[7,20,21]. Finally, the spectral response of the CDCs can be tailored by controlling the SWG parameters or coupling parameters. In particular, phase shifts can be applied to realize resonances within the drop response. Such equivalent “phase-shifted” grating responses have applications for performing temporal operations such as differentiation and Hilbert transformation[2224" target="_self" style="display: inline;">–
A number of techniques exist to implement an optical delay
An ODL that provides time delays for pulses or signals at the same optical carrier is one form of an OTTDL. The conventional architecture of such an OTTDL employs an array of waveguides of different lengths whereby the difference in lengths induces the differential/incremental delay between waveguides (also referred to as taps). For discrete tuning, the different lengths of waveguides can be cascaded using switches or splitters/combiners. To reduce the chip size for integrated implementations, the waveguides are typically arranged with spiral or curvy/serpentine topologies. Indeed, impressive results of 4-bit and 7-bit delays have been obtained in SOI and silicon nitride[26,27].
To minimize or reduce complexities associated with length-variable OTTDLs is to develop an index-variable OTTDL, where true time delay control can be obtained through variation in the group index or propagation velocity in the waveguides. Gasulla and Capmany proposed to exploit the parallelism inherent in multicore fibers to implement an index-variable OTTDL, where the true time delay of each tap can be controlled through designing a proper physical dimension and material doping concentration of each fiber core[28].
The use of SWG waveguides to create a delay or path mismatch in a Mach–Zehnder interferometer was demonstrated recently[29]. We built on this idea and took advantage of the ability to tune the effective index of an SWG waveguide through control of its duty cycle
To investigate the minimum incremental time delay that is possible, we fabricated 2-arm MZIs incorporating SWG waveguides with different duty cycles in each arm, i.e., with a duty cycle difference of
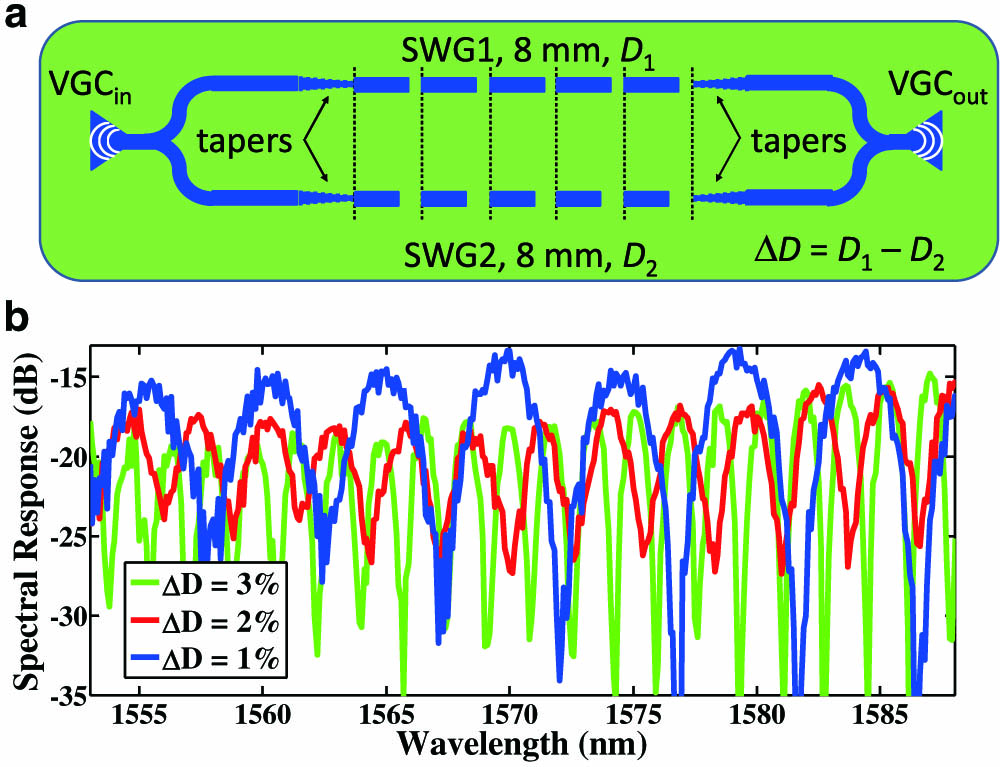
Fig. 7. (a) Schematic of MZI incorporating SWG waveguides with different duty cycles and in each arm. (b) Measured spectral responses of MZIs with , 2%, and 3%.
Next, we fabricated an OTTDL for microwave phase shifting. The structure comprises an array of 4 separate SWG waveguides (a microwave signal modulated on an optical carrier will experience a different phase shift when propagating through the different SWG waveguides) of the same length (8 mm) and where the duty cycles are varied in 10% increments from
The measured RF phase shift as a function of the frequency from the 4 different SWG waveguides is shown in Fig.

Fig. 8. (a) Schematic of OTTDL based on an array of 4 SWG waveguides and experimental setup for microwave phase shift measurements. (b) Measured RF phase shift vs. modulation frequency for an optical carrier at 1565 nm.
We have provided an overview of the recent work on developing wavelength-selective SWG waveguide filters based on MRRs, BGs, and CDCs, as well as index-variable OTTDLs. Since SWG waveguides are based on the same technology and waveguide design as silicon nanowires, they are compatible with existing silicon photonic devices, e.g., switches and modulators. As such, SWG waveguides enhance the available component toolbox for developing integrated MWP systems in SOI. The technological developments described here, along with others in silicon photonics, point to the feasibility of more complex integrated MWP systems that can provide increased functionality and, ultimately, performance.
[1]
[2]
[3]
[4]
[5]
[6]
[7]
[8]
[9]
[10]
[11]
[12]
[13]
[14]
[15]
[16]
[17]
[18]
[19]
[20]
[21]
[22]
[23]
[24]
[25]
[26]
[27]
[28]
[29]
[30]
Lawrence R. Chen. Subwavelength grating waveguide devices in silicon-on-insulators for integrated microwave photonics (Invited Paper)[J]. Chinese Optics Letters, 2017, 15(1): 010004.