Midinfrared optical frequency comb based on difference frequency generation using high repetition rate Er-doped fiber laser with single wall carbon nanotube film
Download: 862次
1. INTRODUCTION
In the midinfrared (MIR) region, there are a variety of absorption spectra of fundamental vibration bands of molecular gases. Such absorption spectra have precise structures, and this region is called the finger print region. Thus MIR spectroscopy allows us to identify and quantify molecular species in a sample.
For the MIR spectroscopy, the Fourier transform infrared spectroscopy with lamp light sources has been generally used. The optical frequency comb based techniques enable high speed molecular sensing with ultrahigh accuracy [1]. Several applications have been investigated in the fields of fundamental research, industries, biomedicine, environmental sensing, and so forth [1,2]. Nowadays, the development of a robust and practical MIR comb is desired to realize highly sensitive and highly accurate molecular identification.
Fiber laser based combs are attractive for their stable, practical, and reliable performances. So far, MIR comb generation pumped by fiber lasers has been demonstrated by several groups. The difference frequency generation (DFG) pumped by Er- [35" target="_self" style="display: inline;">–
Single wall carbon nanotube (SWNT) is one of the attractive devices as the saturable absorber of ultrashort pulse fiber lasers [12–
In this work, we demonstrated MIR comb generation using an Er-doped SWNT fiber laser based system. A high power, ultrashort pulse with high repetition frequency of 161 MHz was generated by an SWNT fiber laser and fiber amplifier. A widely broadened SC was generated with highly nonlinear fiber and high power, ultrashort pulse. The characteristics of the generated SC were examined both experimentally and numerically, and the fiber length was optimized in terms of MIR comb generation. The generated SC was focused onto the nonlinear crystal, and the offset-free MIR comb was generated around the 3 μm wavelength range through DFG. The developed system was an almost all-fiber configuration, and the stable generation of MIR comb was realized.
2. EXPERIMENTAL SETUP AND HIGH POWER, ULTRASHORT PULSE GENERATION
Using the OPO, although we can achieve a high power MIR comb, it needs synchronized feedback control [8–
Figure
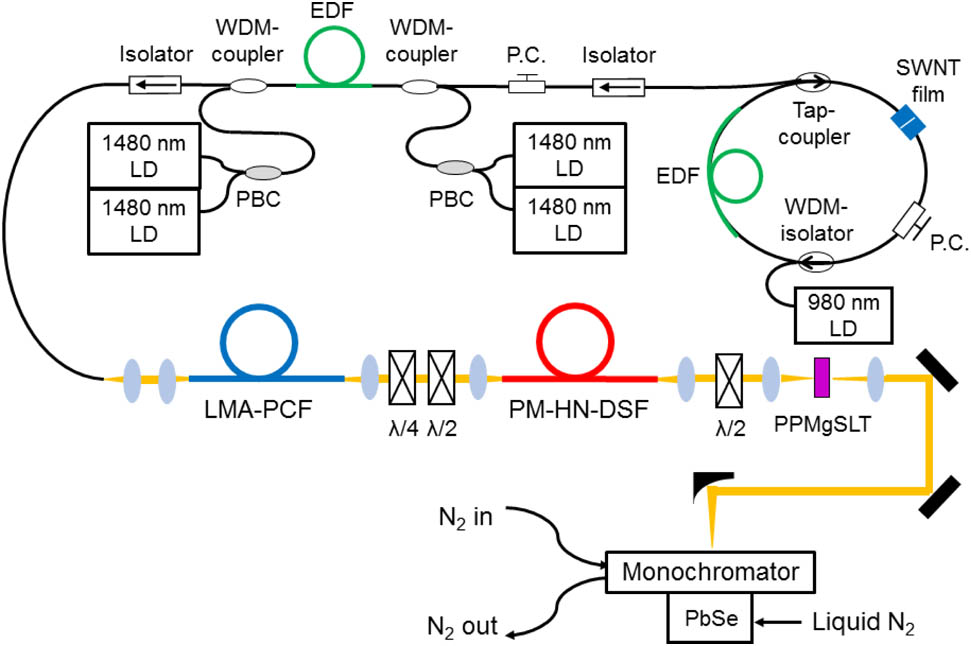
Fig. 1. Experimental setup of MIR comb generation based on Er-doped, ultrashort pulse fiber laser system.
As the seed pulse source, we made passively mode-locked, Er-doped, ultrashort pulse fiber laser with an SWNT saturable absorber [13,14]. The SWNT was synthesized by the laser ablation method, and the diameter of 1.2 nm was obtained selectively. The SWNT was equally dispersed in polyimide liquid, and a free-standing polyimide film was fabricated by water evaporation. The SWNT polyimide film was easily inserted between the fiber connectors inside the fiber laser cavity, and it works as a fast and wideband mode locker.
The fiber laser cavity consists of nonpolarization maintaining single mode fiber devices. In order to achieve the wide comb mode spacing, we made a high repetition rate, ultrashort pulse fiber laser. A 0.5 m length of Er-doped fiber (EDF) pumped by a high power laser diode (LD) at 980 nm wavelength was used as the gain medium. The polarization controller, optical isolator, and output coupler were used to construct the cavity. The net cavity dispersion was
Figure

Fig. 2. Characteristics of output pulse from fiber laser: (a) temporal shape with instantaneous wavelength, (b) optical spectrum, and (c) RF spectra.
The output pulse from the fiber laser was introduced into an EDF amplifier, which consists of high concentration EDF with positive dispersion property. Four high power LDs at 1480 nm wavelength were used as the pump laser. The average power was increased from 11 to 420 mW. Figure
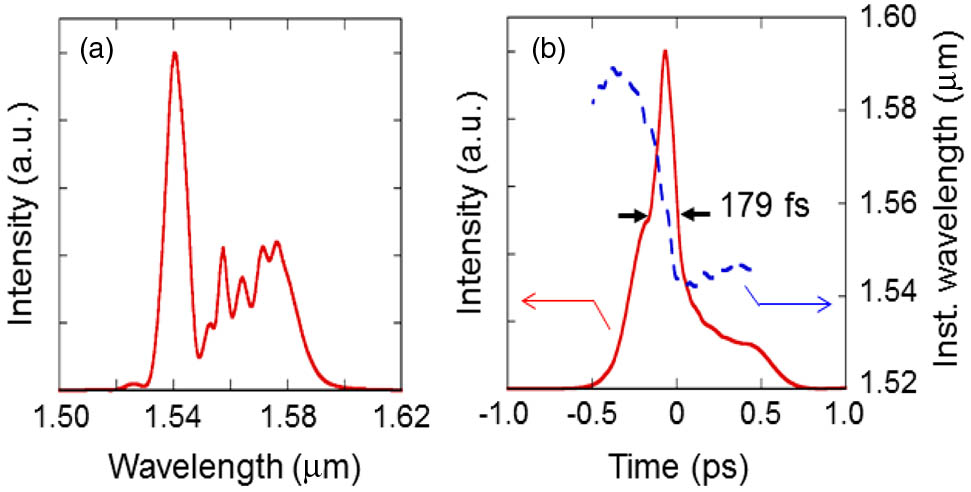
Fig. 3. Characteristics of output pulse from fiber amplifier: (a) optical spectrum and (b) temporal shape with instantaneous wavelength.
Then we applied dispersion compensation using a large mode field area photonic crystal fiber (LMA-PCF, LMA30). Using the LMA-PCF, we demonstrated dispersion compensation effectively while suppressing the nonlinear effect. The magnitude of second order dispersion
Figure
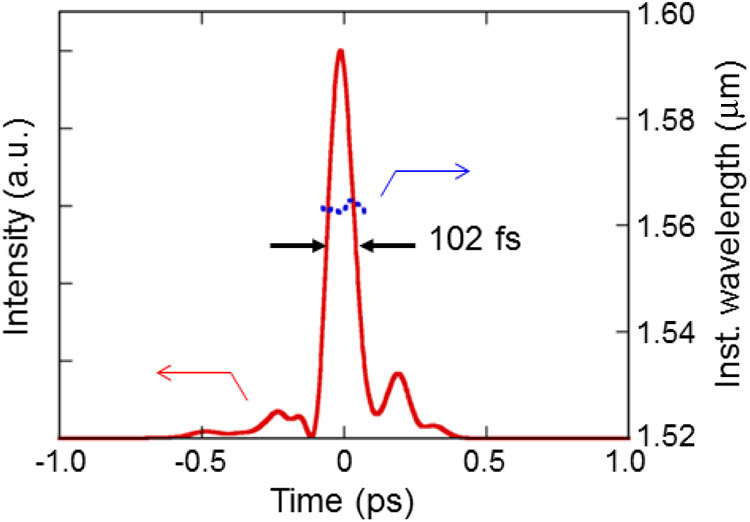
Fig. 4. Temporal shape and instantaneous wavelength of dispersion compensated ultrashort pulse with LMA-PCF.
3. GENERATION OF SC AND MIR COMB
Next, in order to generate the optical frequency comb at the MIR region by DFG, we generated SC. A polarization maintaining, highly nonlinear, dispersion shifted fiber (PM-HN-DSF) was used as the nonlinear device [21]. The zero-dispersion wavelength was 1.55 μm, and the mode-field diameter was 3.0 μm. The magnitude of the dispersion parameter
In order to examine the optimum fiber length, we demonstrated the numerical analysis of SC generation. Figure

Fig. 5. (a), (c), and (e) optical spectra and (b), (d), and (f) temporal shape of output pulse from highly nonlinear fiber for the length of 10, 20, and 30 cm.
Figure
Figure
Next, we examined the coherence properties of the generated SC by the balanced heterodyne RF beat measurement. Figure
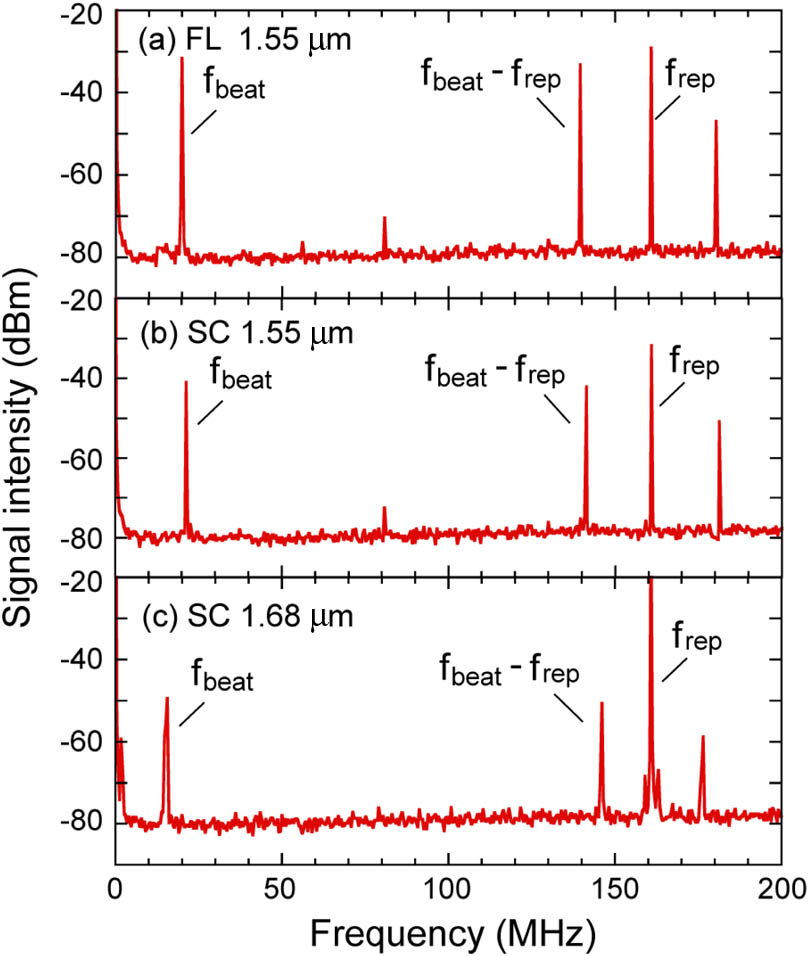
Fig. 8. Observed RF beat notes between cw-LD and (a) fiber laser at 1550 nm, (b) SC at 1550 nm, and (c) SC at 1680 nm.
Next, in order to investigate the feasibility of MIR comb generation using the generated SC, we observed temporal distribution of spectral components using the cross-correlation FROG (X-FROG) [20,25,26]. The generated SC was introduced into a sum frequency generation type autocorrelation FROG system. A long pass filter was used in one arm of the FROG, and the longer wavelength side was picked off and used as the probe pulse. A 1 mm thick BaB2O4 crystal was used as the
Figure
Next, we focused the generated SC into a nonlinear crystal. In this work, we used fan-out type periodically poled MgSLT (PPMgSLT). The PPMgSLT was designed to generate an MIR comb at the 3.0–5.0 μm region by a 1.3–1.8 μm near-infrared pulse. The length and thickness of the crystal were 11 and 1.0 mm, respectively.
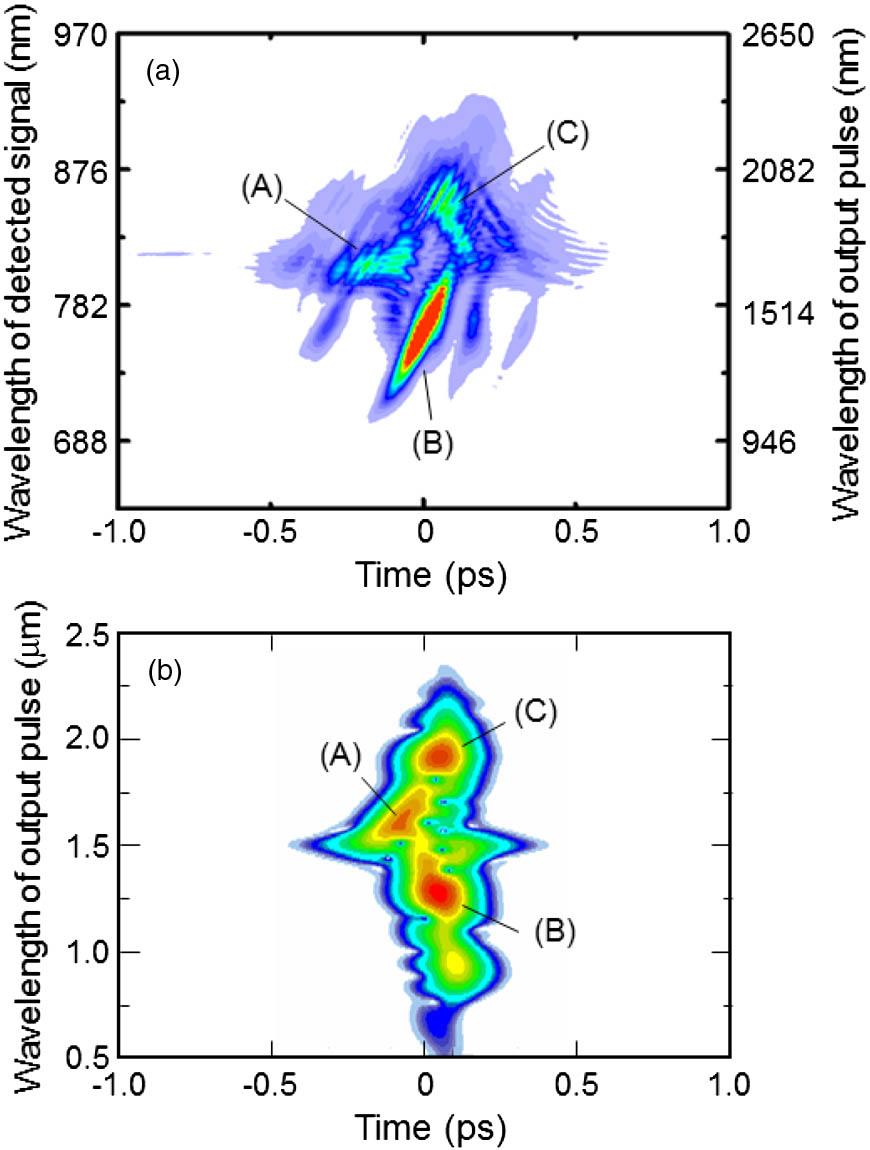
Fig. 9. Spectrogram of generated SC in 20 cm of highly nonlinear fiber; (a) experimentally observed spectrogram with X-FROG, and (b) numerically obtained spectrogram with PG-FROG.
Figure
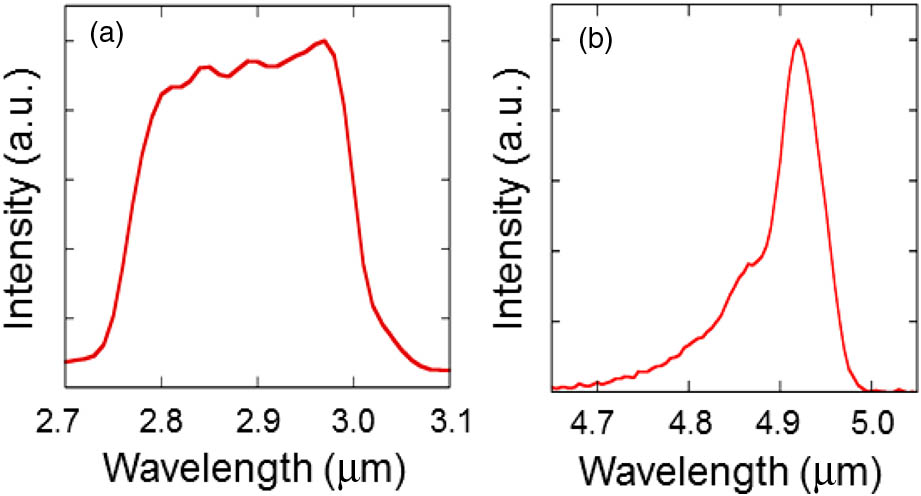
Fig. 10. Observed optical spectra of generated MIR comb at wavelengths of (a) 2.9 and (b) 4.9 μm.
Thanks to the high coherence property of the SC generated in a short PM-HN-DSF, the high temporal coherence of obtained MIR comb is expected. Recently, we directly confirmed the high coherence property of the MIR comb pumped with a coherent SC generated in a Yb-doped fiber laser system [27].
4. CONCLUSION
In conclusion, we demonstrated MIR optical frequency comb generation at the 3.0 μm region by DFG pumped by a high power fiber laser SC. A soliton mode-locked, 161 MHz, high repetition rate EDF laser was constructed using SWNT film. Then a 102 fs, 17 kW, high power, ultrashort pulse was generated using fiber amplifier and large mode field area fiber. Considering the numerical result, a 1.1–2.2 μm wideband SC was generated for the DFG. The spectrogram of generated SC was examined both experimentally and numerically, and the temporal distribution of spectral components was confirmed. Then the generated SC was focused into the nonlinear crystal, and the stable generation of an MIR comb around 2.9 and 4.9 μm wavelength regions was realized. The developed system consists of almost all-fiber devices, and it is useful for practical applications. Further power scaling is under investigation.
5 Acknowledgment
Acknowledgment. This research is partially supported by the SENTAN program “Development of Systems and Technology for Advanced Measurement and Analysis” of the JST, and the AMED.
Article Outline
M. Tsuzuki, L. Jin, M. Yamanaka, V. Sonnenchein, H. Tomita, A. Sato, T. Ohara, Y. Sakakibara, E. Omoda, H. Kataura, T. Iguchi, N. Nishizawa. Midinfrared optical frequency comb based on difference frequency generation using high repetition rate Er-doped fiber laser with single wall carbon nanotube film[J]. Photonics Research, 2016, 4(6): 06000313.