超快激光调控晶体形核与生长过程研究进展
下载: 1335次特邀综述
Significance Crystallization has applications in biomedicine, structural analysis, and other related fields. For example, single crystal X-ray diffraction (XRD) is a common method for the structural analysis of biomacromolecules. Polymorph crystallization is also of significance in the pharmaceutical industry. These applications require the number, size, and polymorph of the crystals to be determined. Conventionally, crystals are obtained by evaporation of a solution or via a batch cooling process. However, the complex nature of the crystallization process means that precise control of crystallization is difficult.
The crystallization process consists of two main stages: nucleation and crystal growth. When the concentration of a solute exceeds its solubility, the supersaturated solution is in a metastable zone. When the solute concentration reaches the supersaturation limit, nucleation occurs. The nucleus will then grow into larger crystals when the concentration drops back to the solubility level.
In recent years, various methods have been studied for controlling crystal nucleation and growth processes, including those involving lasers, ultrasonics, and electromagnetic fields (
Progress The ultrafast laser-controlled crystallization process can be categorized into several different types depending on stage of crystallization where the laser is involved (
After the crystal nucleus dissolves out from the solution, laser interaction with the crystals or the surrounding solution can influence the crystal growth process. Laser irradiation of the solution can be performed to change the growth rate of crystals through a laser trapping phenomenon. For some organic materials, laser trapping increases the concentration at the focal point and accelerates the crystal growth. For some other materials, such as proteins, the electromagnetic field will keep the molecules and clusters in a low energy state and restrain the crystal growth. In addition to the control of the entire crystal growth rate, the growth of a specific crystal face can also be promoted. Ultrafast laser ablation on a crystal surface alters the growth mode and enhances the growth speed of the specific crystal face(
Ultrafast laser ablation has high precision and has a limited thermal effect on the surrounding materials because of the nonlinear absorption effect and non-thermal ablation process. Therefore, it is suitable for the processing of thermally sensitive materials, including proteins, amino acids, and other biomaterials. Arbitrary micropatterns such as microarrays can be achieved on the surface of single protein crystals without thermal damage using femtosecond laser processing. Ultrafast laser cleaving of protein crystals can be performed to fabricate crystal seeds with high quality. Micropatterning on single crystals has potential applications in the fabrication of biological devices.
Conclusion and Prospect In conclusion, ultrafast laser can be used to control the nucleation and crystal growth processes. This approach is applicable for many biomedical fields because it can control crystallization and has limited thermal effects. Ultrafast laser control of the crystallization process still poses challenges such as lack of mechanism understanding and limits in practical applications. Future studies on its mechanism and cross-disciplinary collaboration will enhance the significance and application prospect of this method.
1 引言
物质在溶液中的结晶涉及生物、材料、医学等研究领域,可以被应用于生物制药、大分子结构分析等方面。单晶X射线衍射(XRD)是生物大分子结构分析中最常用的手段之一,需要通过溶液中结晶的方法得到高质量的单晶。传统的方法是通过调节环境温度和湿度,使待结晶物质的过饱和溶液缓慢蒸发,直到溶质析出形核,进而生长成所需的晶体。由于结晶机理的复杂性,结晶过程对环境的要求极为苛刻,在结晶过程中控制结晶的大小、形状、晶型等存在一定困难[1]。针对这一问题,一些外场辅助结晶的方法被提出,如在结晶过程中辅以激光、超声、电磁场、微波、剪切力等,可对晶体的形核与生长具有一定的调控作用[2-10]。超快激光是一种调控效果好、灵活性高、对材料损伤小的调控结晶手段。超快激光是指脉宽在飞秒(10-15 s)到皮秒(10-12 s)量级的脉冲激光,具有超快、超强的特点,可适用的材料范围广,加工精度高,热影响区域小,已被广泛应用于微纳加工、生物医药等领域[11-14]。近年来,超快激光在材料结晶领域的应用得到了相关研究人员的关注。
目前,超快激光调控晶体形核生长的方法主要可以分为超快激光诱导结晶形核、控制晶体生长过程以及晶面图案化加工这三类。如

图 1. 超快激光调控晶体形核与生长的方法及其应用
Fig. 1. Methods and applications of ultrafast laser induced nucleation and growth
2 超快激光诱导结晶形核
2.1 诱导结晶形核的主要方法
溶液中的结晶可以分为形核和生长两个主要过程。形核过程是指溶质分子从过饱和溶液中析出形成晶核的过程,而生长是指已经产生的晶核在溶液中继续长大得到所需晶体的过程。其中形核过程对于结晶结果中的晶体数量、晶型、晶体质量有很大影响。为了实现对结晶形核过程的控制,一些外场辅助的诱导结晶形核方法得到了大量研究,如
表 1. 调控晶体形核的主要方法分类
Table 1. Methods of controlling the nucleation process
|
超快激光具有超快超强的特点,因此其与溶液的作用机理与连续激光或纳秒激光不同。物质对超快激光具有多光子吸收效应,超快激光的能量可以被不同种类的基底、溶液吸收。超快激光与溶液作用过程中存在光电场作用、局部热效应以及冲击波、空化气泡等现象,因此与溶液的作用过程更加复杂,对结晶形核具有独特的调控作用。
根据超快激光的激光参数和作用机制,超快激光诱导结晶形核的方法可以分为
![激光诱导结晶形核的方法和实例。(a)激光在基底表面产生热效应诱导形核;(b)飞秒激光诱导钙钛矿结晶,图中标尺为50 μm[27];(c)激光在溶液中形成空化气泡并促进结晶形核;(d)飞秒激光诱导溶液中蛋白质结晶[35];(e)非光化学激光诱导结晶形核;(f)不同辐照时间下纳秒激光诱导氯化钾溶液结晶,图中标尺为0.5 cm[3]](/richHtml/zgjg/2021/48/2/0202020/img_2.jpg)
图 2. 激光诱导结晶形核的方法和实例。(a)激光在基底表面产生热效应诱导形核;(b)飞秒激光诱导钙钛矿结晶,图中标尺为50 μm[27];(c)激光在溶液中形成空化气泡并促进结晶形核;(d)飞秒激光诱导溶液中蛋白质结晶[35];(e)非光化学激光诱导结晶形核;(f)不同辐照时间下纳秒激光诱导氯化钾溶液结晶,图中标尺为0.5 cm[3]
Fig. 2. Methods and examples of laser induced nucleation. (a) Laser induced crystallization by local heating of the substrate; (b) femtosecond laser induced crystallization of perovskite, and the scale bar is 50 μm[27]; (c) laser induced cavitation bubbles and following nucleation; (d) femtosecond laser induced nucleation of hen egg white lysozyme (HEWL)[35]; (e) non-photochemical laser induced nucleation
超快激光不仅可以用于调控结晶形核的数量和大小,近年来,人们发现超快激光可以诱导不同晶型的形核。由于一些药物分子不同的晶型会影响药物的物理化学性质,因此对磺胺噻唑、扑热息痛等有机物的形核过程进行调控在生物制药领域具有一定的应用价值[44-48]。除了温度梯度、超声等外场辅助手段以外,超快激光诱导形核方法也被用于提高某些特定晶型的比例。如
![时间整形飞秒激光诱导扑热息痛结晶形核[33]。(a)飞秒激光作用不同时间后的结晶形核结果;(b)最优参数下晶体的长宽比分布;(c)飞秒激光与溶液作用产生空化气泡的超快过程观测结果;(d)飞秒激光在溶液中产生空化气泡诱导结晶形核过程的机理示意图](/richHtml/zgjg/2021/48/2/0202020/img_3.jpg)
图 3. 时间整形飞秒激光诱导扑热息痛结晶形核[33]。(a)飞秒激光作用不同时间后的结晶形核结果;(b)最优参数下晶体的长宽比分布;(c)飞秒激光与溶液作用产生空化气泡的超快过程观测结果;(d)飞秒激光在溶液中产生空化气泡诱导结晶形核过程的机理示意图
Fig. 3. Spatiotemporally shaped femtosecond laser induced nucleation of paracetamol[33]. (a) Femtosecond laser induced nucleation of paracetamol after different time; (b) aspect ratio of the crystal at the optimal parameters ; (c) time-resolved images of femtosecond laser induced cavitation bubbles; (d) schematics of controlled nucleation caused by laser induced cavitation bubbles
2.2 超快激光诱导结晶形核的机理
通过对超快激光诱导结晶形核过程进行超快观测和理论分析,可以对其诱导形核的机理有更深入的理解。超快激光与材料的作用是一个跨时间、空间尺度的非线性非平衡过程,激光与材料作用过程中存在能量吸收、光电场影响等多种效应的共同作用,其诱导的结晶形核也存在多种机理的耦合。目前,对于激光诱导结晶的机理有多种解释,根据使用的激光参数和材料体系不同,这些作用机理主要可以分为空化气泡和非光化学诱导结晶两大类。从空化气泡角度出发的解释认为,超快激光可以被多光子吸收,溶液吸收飞秒激光能量后产生冲击波和空化气泡[49-50],在此过程中,冲击波和空化气泡可以在局部产生扰动,改变局部的溶质浓度。当溶质浓度高于过饱和线时,就会析出形核。一些研究通过对超快激光与溶液作用过程的观测与对溶质的荧光标记,证明了激光诱导空化气泡附近的溶质浓度升高[37-39];同时,由于空化气泡与溶液接触界面上表面能的变化降低了形核势垒,溶质更容易在气液界面上析出形核,从而对结晶形核起到了促进作用。这一机理在超声辅助形核、材料界面异相形核的研究中也有相关讨论[7, 51-52]。除了空化气泡的作用外,在非光化学诱导形核中,光学克尔效应、电场极化,以及溶液中杂质对激光的吸收效应等,也对结晶形核有着调控作用[3]。控制变量的实验可以分别证明这些效应对形核的作用:有人通过倏释波诱导形核证明了单独的光电场对结晶形核具有促进作用[17];有人在控制激光偏振的实验中发现,使用线偏振和圆偏振的激光可以诱导磺胺噻唑等有机物形成不同晶型的晶核,证明了极化方向对形核的影响[26];激光诱导甘氨酸的结晶形核率与溶液中的杂质含量相关,这说明激光对溶液中杂质颗粒的加热也是非光化学激光诱导形核的原因之一[25]。
尽管超快激光诱导结晶形核过程涉及多种机理,目前也还没有统一的解释,但这类方法都可以总结为,激光与溶液的作用提高了局部的溶质浓度或降低了溶解度,从而在局部发生形核。基于这一原理,超快激光诱导结晶形核具有高空间选择性的特点;同时,这种方法可以在溶液还未发生自发形核的情况下,在局部形成少量晶核,这样的晶核在长大后可以获得体积更大、质量更好的单晶。
3 超快激光调控晶体的生长过程
3.1 超快激光调控晶体的生长过程
超快激光除了可以被应用于诱导结晶形核外,也可以被应用于对晶体的生长过程进行调控。晶核形成后,随着溶液浓度从过饱和状态回到饱和状态,以及溶剂的继续蒸发,溶液中的分子会进一步析出,并倾向于在已经成核的晶体表面沉积,实现晶体的生长。晶体的生长过程会影响最终获得的晶体的大小、形状、晶体质量等。在诸如蛋白质结构分析等对晶体体积和质量要求较高的应用场景中,对晶体生长的控制有助于得到符合要求的单晶,因此激光调控晶体生长的方法也得到了研究,如
表 2. 激光调控晶体生长方法的主要分类
Table 2. Main categories of laser controlled crystal growth methods
|
超快激光主要可以通过对溶液或晶体的作用实现不同的晶体生长调控效果。一种方式如
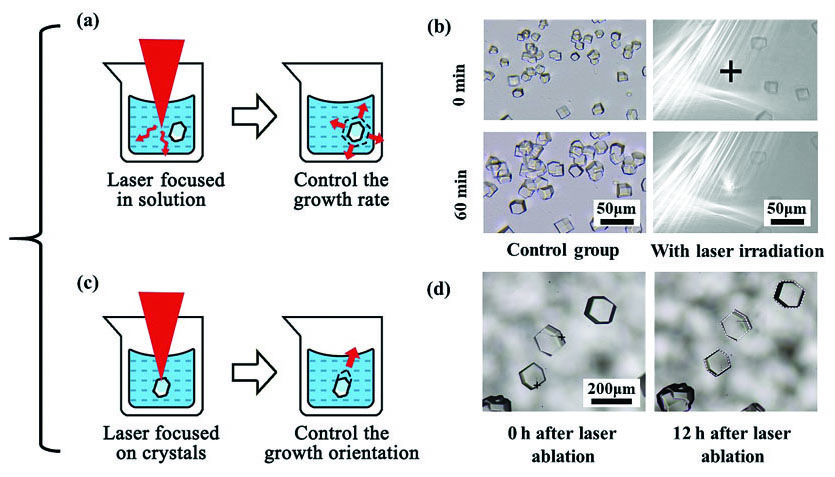
图 5. 激光调控晶体生长速度的方法和实例。(a)激光作用于溶液内部调控晶体的整体生长速度;(b)飞秒激光抑制蛋白质晶体的生长速度;(c)激光作用于晶体表面控制晶体的生长取向;(d)飞秒激光烧蚀促进某一晶面的生长
Fig. 5. Methods and examples of laser controlled crystal growth. (a) Control of the growth rate of crystals by laser irradiation in the solution; (b) femtosecond laser controlled growth of HEWL compared with non-irradiated crystals; (c) control of the growth orientation by laser ablation of crystal surface; (d) promotion of the growth rate of one crystal surface by femtosecond laser ablation
3.2 超快激光调控晶体生长过程的机理
通过对晶体生长过程和结晶结果进行观测,可以对超快激光调控晶体生长过程的机理进行解释。如

图 6. 激光调控晶体生长过程的不同方法及其机理
Fig. 6. Methods and mechanisms of laser controlled crystal growth
超快激光在蛋白质晶体表面的烧蚀加工也可以调控激光烧蚀晶面的生长速度,这是因为在晶体表面的烧蚀形成了螺旋位错,改变了晶体的生长模式。由于一般的晶体生长模式为逐层生长,在一层晶体生长到足够大时,上一层晶体才会开始生长,因此速度较慢,而螺旋生长模式不受这一限制[55]。对于生长中的晶面的微分干涉显微成像表征,也证明了螺旋生长的速度要快于逐层生长的速度[63]。
4 超快激光图案化加工晶体表面
除了可以对晶体形核与生长过程进行调控外,超快激光还可以应用于晶体的微纳加工,获得理想的晶体形状,或在晶体表面实现图案化的微纳结构。超快激光对于溶液中晶体的微纳加工具有多种应用前景,可以被应用于晶种的制备以及微器件的制造等。由于自发形核的晶体可能存在缺陷,利用超快激光非热烧蚀可以对已有晶体进行切割,从而得到质量更好的晶种。另一种方法是烧蚀产生材料溅射,在溶液中重新获得新的晶种,从而继续生长得到质量较好的单晶[57-61]。除了直接加工晶体,超快激光加工方法还可以通过对基底的加工来调控结晶的结果。由于激光可以通过微纳加工和表面改性来改变表面的浸润性能[64-65],因此可以在基底上加工出微纳结构来控制溶液在其表面的亲疏水性分布,使溶液在目标位置沉积并蒸发结晶[66-67]。
对于晶体表面的图案化加工,尤其是对生物大分子晶体的加工,目前仍然存在着困难。因为分子晶体的结晶主要依靠分子间作用力,结合能力较弱,在外场作用下容易发生破碎,而生物材料又容易受到热影响而改性,因此很多微纳加工手段受到了限制。超快激光以加工精度高、热影响区域小的优势可以被应用于蛋白质等一些对热效应较为敏感的材料的加工[68-69]。本研究团队采用超快激光在蛋白质晶体表面进行了加工,
![飞秒激光烧蚀加工蛋白质晶体的结果和过程示意图。(a)(b)飞秒激光在鸡蛋白溶菌酶晶体表面加工图案化微纳结构[70];(c)飞秒激光电离电介质材料的主要机理;(d)飞秒激光与蛋白质晶体作用示意图](/richHtml/zgjg/2021/48/2/0202020/img_7.jpg)
图 7. 飞秒激光烧蚀加工蛋白质晶体的结果和过程示意图。(a)(b)飞秒激光在鸡蛋白溶菌酶晶体表面加工图案化微纳结构[70];(c)飞秒激光电离电介质材料的主要机理;(d)飞秒激光与蛋白质晶体作用示意图
Fig. 7. Ablation results and schematics of femtosecond laser processing on protein crystals. (a)(b) Femtosecond laser processing patterned micro-nano structures on the surface of protein crystal[70]; (c) main mechanisms of femtosecond laser ionization of dielectric materials; (d) schematics of femtosecond laser interaction with protein crystals
超快激光与物质的作用是一个非线性、非平衡的过程。以蛋白质晶体为例,蛋白质属于电介质材料,其带隙较宽,对于红外和可见激光无法吸收单光子能量。而超快激光由于峰值功率极高,可以通过非线性效应被材料吸收[71],可以对一些特殊材料,尤其是生物材料和透明材料进行非热加工,而且可以实现超衍射极限的加工精度,热影响区小[69-70,72-77]。
5 超快激光调控结晶应用前景的展望
超快激光调控晶体形核生长过程与蛋白质晶体学的结合有着广阔的应用前景。蛋白质晶体学是生物学中的重要分支学科,主要研究蛋白质晶体的结构及性质。单晶XRD是蛋白质结构解析的主要方法之一,同步辐射光源的引入可以显著提升XRD技术的精度,这种方法在今后还将有更大的发展潜力。单晶XRD的前提是得到质量较高的单晶,通过超快激光调控晶体的形核与生长,不仅可以实现难结晶物质的结晶,还可以调控晶体的数量、大小、形状,在获得高质量单晶方面具有重要的应用价值。
超快激光调控结晶还可以被应用于生物制药等领域。由于一些有机药物存在不同的晶型,而不同晶型的物理化学性质不同,会影响药物的溶解吸收等过程,因此在制药中会倾向于得到某些特定晶型的结晶。超快激光调控结晶形核在这一领域的应用将会提高所需晶型的产率。另外,近年来纳米药物以其独特的物理化学性质和吸收率得到了许多研究人员的关注,研究人员将超快激光加工晶体材料的方法应用于制备纳米级药物晶体颗粒[79]。
随着材料技术和生物医药的发展,生物材料基的微器件得到了越来越多的研究和应用[80-82],如蛋白质/DNA芯片[83-84]、生物传感器[85]等。超快激光微纳加工技术的发展,尤其是超快激光在生物材料晶体表面的微纳米图案化加工,为生物材料基微器件的制备奠定了基础。例如,超快激光在蛋白质晶体材料表面的微结构加工,可以被应用于蛋白质基微器件的制备,包括细胞支架[86-88]、药物输运[89]、生物传感器[90-91]等的制备。此外,超快激光诱导的选择性结晶在蛋白质芯片、生物检测等方面也具有一定的应用价值[92-94]。
超快激光调控晶体形核与生长的应用还存在着一些挑战,与传统的结晶方法以及超声等辅助手段相比,超快激光还存在成本高、效率低等问题,从而限制了它的大规模工业应用。随着多焦点阵列的并行加工等高效加工方法的发展,激光加工的效率问题将会得到改善[95]。从另一角度来看,相比工业领域,超快激光调控结晶在前沿科研领域的应用优势更加显著,具有高空间选择性、适用材料范围广等其他结晶方法所不具备的优势。超快激光是制造领域的先进技术,它与生物、材料等学科中前沿研究的交叉融合,在未来的研究中可能产生新的科研突破。
6 结束语
本文综述了超快激光在调控晶体形核和生长方面的研究进展:在诱导形核方面,已经实现了超快激光对结晶形核过程的调控,通过改变激光参数可以提高结晶率以及调控结晶的数量、大小和晶型;在控制晶体生长方面,超快激光可以通过对溶液或晶体的作用,调控晶体整体或某一晶面的生长速度。另外,采用超快激光对晶体直接进行加工具有高精度、低热影响的优势。目前,超快激光诱导晶体形核与生长仍然有一些问题需要解决:一方面,超快激光调控晶体形核生长的具体机理还没有得到很好的解释,这是因为超快激光与材料的作用过程存在多种机理的耦合,给激光诱导结晶过程的机理研究和参数设计带来了挑战;另一方面,超快激光调控晶体形核生长方法的应用受到成本和效率的限制,前沿的跨学科研究也存在一定局限。
总而言之,超快激光调控晶体形核与生长过程的研究具有重要价值,对超快激光与材料作用机理的深入探索以及对更多跨学科交叉应用的研究,将会使超快激光调控晶体形核与生长的技术取得新进展,在晶体学、大分子结构分析、生物制药等科研和工程领域发挥更多作用。
[1] McPherson A, GaviraJ A. Introduction to protein crystallization[J]. Acta Crystallogr Section F, 2014, 70(1): 2-20.
[2] Yoshikawa H Y, Murai R, Adachi H, et al. Laser ablation for protein crystal nucleation and seeding[J]. Chemical Society Reviews, 2014, 43(7): 2147-2158.
[3] Alexander A J, Camp P J. Non-photochemical laser-induced nucleation[J]. The Journal of Chemical Physics, 2019, 150(4): 040901.
[4] Liu Y, Alexander A J. Supersaturation dependence of glycine polymorphism using laser-induced nucleation, sonocrystallization and nucleation by mechanical shock[J]. Physical Chemistry Chemical Physics, 2017, 19(29): 19386-19392.
[5] Mori Y, Maruyama M, Takahashi Y, et al. Selective crystallization of metastable phase of acetaminophen by ultrasonic irradiation[J]. Applied Physics Express, 2015, 8(6): 065501.
[6] Su C S, Liao C Y, Jheng W D. Particlesize control and crystal habit modification of phenacetin using ultrasonic crystallization[J]. Chemical Engineering & Technology, 2015, 38(1): 181-186.
[7] Bhangu S K, Ashokkumar M, Lee J. Ultrasound assisted crystallization of paracetamol: crystal size distribution and polymorph control[J]. Crystal Growth & Design, 2016, 16(4): 1934-1941.
[8] Sudha C, Sivanarendiran R, Srinivasan K. Influence of magnetic field on the nucleation rate control of mono paracetamol[J]. Crystal Research and Technology, 2015, 50(3): 230-235.
[9] Mohammed M. SyedM F, Bhatt M J, et al. Rapid and selective crystallization of acetaminophen using metal-assisted and microwave-accelerated evaporative crystallization[J]. Nano Biomedicine and Engineering, 2012, 4(1): 35-40.
[10] Sun J K, Sobolev Y I, Zhang W Y, et al. Enhancing crystal growth using polyelectrolyte solutions and shear flow[J]. Nature, 2020, 579(7797): 73-79.
[11] 姜澜, 李丽珊, 王素梅, 等. 飞秒激光与宽禁带物质相互作用过程中光子-电子-声子之间的微能量传导I: 光子吸收过程[J]. 中国激光, 2009, 36(4): 779-789.
Jiang L, Li L S, Wang S M, et al. Microscopic energy transport through photon-electron-phonon interactions during ultrashort laser ablation of wide bandgap materials Part I: photon absorption[J]. Chinese Journal of Lasers, 2009, 36(5): 779-789.
[12] 钟敏霖, 范培迅. 激光纳米制造技术的应用[J]. 中国激光, 2011, 38(6): 0601001.
[13] Jiang L. WangA D, Li B, et al. Electrons dynamics control by shaping femtosecond laser pulses in micro/nanofabrication: modeling, method, measurement and application[J]. Light Science & Applications, 2018, 7: 17134.
[14] 史杨, 许兵, 吴东, 等. 飞秒激光直写技术制备功能化微流控芯片研究进展[J]. 中国激光, 2019, 46(10): 1000001.
[15] Alexander A J, Camp P J. Single pulse, single crystal laser-induced nucleation of potassium chloride[J]. Crystal Growth & Design, 2009, 9(2): 958-963.
[16] Duffus C, Camp P J, Alexander A J. Spatial control of crystal nucleation in agarose gel[J]. Journal of the American Chemical Society, 2009, 131(33): 11676-11677.
[17] Ward M R, Rae A, Alexander A J. Nonphotochemical laser-induced crystal nucleation by an evanescent wave[J]. Crystal Growth & Design, 2015, 15(9): 4600-4605.
[18] Shilpa T. Small and macromolecules crystallization induced by focused ultrafast laser[J]. Proceedings of the Indian National Science Academy, 2015, 81(2): 517-523.
[19] Kacker R, Dhingra S, Irimia D, et al. Multiparameter investigation of laser-induced nucleation of supersaturated aqueous KCl solutions[J]. Crystal Growth & Design, 2018, 18(1): 312-317.
[20] Mirsaleh-Kohan N, Fischer A, Graves B, et al. Laser shock wave induced crystallization[J]. Crystal Growth & Design, 2017, 17(2): 576-581.
[21] Barber E R. Kinney N L H, Alexander A J. Pulsed laser-induced nucleation of sodium chlorate at high energy densities[J]. Crystal Growth & Design, 2019, 19(12): 7106-7111.
[22] Yoshikawa H Y, Hosokawa Y, Masuhara H. Explosive crystallization of urea triggered by focused femtosecond laser irradiation[J]. Japanese Journal of Applied Physics, 2006, 45(1): L23-L26.
[23] Chou S S, Swartzentruber B S, Janish M T, et al. Laser direct write synthesis of lead halide perovskites[J]. The Journal of Physical Chemistry Letters, 2016, 7(19): 3736-3741.
[24] Jeon T, Jin H M, Lee S H, et al. Laser crystallization of organic-inorganic hybrid perovskite solar cells[J]. ACS Nano, 2016, 10(8): 7907-7914.
[25] Javid N, Kendall T, Burns I S, et al. Filtration suppresses laser-induced nucleation of glycine in aqueous solutions[J]. Crystal Growth & Design, 2016, 16(8): 4196-4202.
[26] Li W J, Ikni A, Scouflaire P, et al. Non-photochemical laser-induced nucleation of sulfathiazole in a water/ethanol mixture[J]. Crystal Growth & Design, 2016, 16(5): 2514-2526.
[27] Arciniegas M P, Castelli A, Piazza S, et al. Laser-induced localized growth of methylammonium lead halide perovskite nano- and microcrystals on substrates[J]. Advanced Functional Materials, 2017, 27(34): 1701613.
[28] Liu Y, Ward M R, Alexander A J. Polarization independence of laser-induced nucleation in supersaturated aqueous urea solutions[J]. Physical Chemistry Chemical Physics, 2017, 19(5): 3464-3467.
[29] Liu T H, Yuyama K I, Hiramatsu T, et al. Femtosecond-laser-enhanced amyloid fibril formation of insulin[J]. Langmuir, 2017, 33(33): 8311-8318.
[30] Tasnim T, Goh A, Gowayed O, et al. Dendritic growth of glycine from nonphotochemical laser-induced nucleation of supersaturated aqueous solutions in agarose gels[J]. Crystal Growth & Design, 2018, 18(10): 5927-5933.
[31] Yamamoto Y, Nishimura Y, Tokonami S, et al. Macroscopically anisotropic structures produced by light-induced solvothermal assembly of porphyrin dimers[J]. Scientific Reports, 2018, 8: 11108.
[32] Walton F, Wynne K. Using optical tweezing to control phase separation and nucleation near a liquid-liquid critical point[J]. Soft Matter, 2019, 15(41): 8279-8289.
[33] Wang S B, Wang S M, Jiang L, et al. Polymorph-controlled crystallization of acetaminophen through femtosecond laser irradiation[J]. Crystal Growth & Design, 2019, 19(6): 3265-3271.
[34] Yuyama K I, Marcelis L, Su P M, et al. Photocontrolled supramolecular assembling of azobenzene-based biscalix[4]arenes upon starting and stopping laser trapping[J]. Langmuir, 2017, 33(3): 755-763.
[35] Adachi H, Takano K, Hosokawa Y, et al. Laser irradiated growth of protein crystal[J]. Japanese Journal of Applied Physics, 2003, 42: L798-L800.
[36] Nakamura K, Sora Y, Yoshikawa H Y, et al. Femtosecond laser-induced crystallization of protein in gel medium[J]. Applied Surface Science, 2007, 253(15): 6425-6429.
[37] Yoshikawa H Y, Murai R, Sugiyama S, et al. Femtosecond laser-induced nucleation of protein in agarose gel[J]. Journal of Crystal Growth, 2009, 311(3): 956-959.
[38] Murai R, Yoshikawa H Y, Takahashi Y, et al. Enhancement of femtosecond laser-induced nucleation of protein in a gel solution[J]. Applied Physics Letters, 2010, 96(4): 043702.
[39] Iefuji N, Murai R, Maruyama M, et al. Laser-induced nucleation in protein crystallization: local increase in protein concentration induced by femtosecond laser irradiation[J]. Journal of Crystal Growth, 2011, 318(1): 741-744.
[40] Sugiyama T, Yuyama K, Masuhara H. Laser trapping chemistry: from polymer assembly to amino acid crystallization[J]. Accounts of Chemical Research, 2012, 45(11): 1946-1954.
[41] Yuyama K I, Chang K D, Tu J R, et al. Rapid localized crystallization of lysozyme by laser trapping[J]. Physical Chemistry Chemical Physics: PCCP, 2018, 20(9): 6034-6039.
[42] Nabetani Y, Yoshikawa H, Grimsdale A C, et al. Effects of optical trapping and liquid surface deformation on the laser microdeposition of a polymer assembly in solution[J]. Langmuir, 2007, 23(12): 6725-6729.
[43] Ruecroft G, Hipkiss D, Ly T, et al. Sonocrystallization: the use of ultrasound for improved industrial crystallization[J]. Organic Process Research & Development, 2005, 9(6): 923-932.
[44] Munroe Á, Rasmuson Å C, Hodnett B K, et al. Relative stabilities of the five polymorphs of sulfathiazole[J]. Crystal Growth & Design, 2012, 12(6): 2825-2835.
[45] Agnew L R, Cruickshank D L. McGlone T, et al. Controlled production of the elusive metastable form II of acetaminophen (paracetamol): a fully scalable templating approach in a cooling environment[J]. Chemical Communications, 2016, 52(46): 7368-7371.
[46] Higashi K, Ueda K, Moribe K. Recent progress of structural study of polymorphic pharmaceutical drugs[J]. Advanced Drug Delivery Reviews, 2017, 117: 71-85.
[47] Hao X, Liu J F, Luo H Y, et al. Crystal structure optimization and Gibbs free energy comparison of five sulfathiazole polymorphs by the embedded fragment QM method at the DFT level[J]. Crystals, 2019, 9(5): 256.
[48] Song S, Wang L, Yao C L, et al. Crystallization of sulfathiazole in gel: polymorph selectivity and cross-nucleation[J]. Crystal Growth & Design, 2020, 20(1): 9-16.
[49] Faccio D, Tamošauskas G, Rubino E, et al. Cavitation dynamics and directional microbubble ejection induced by intense femtosecond laser pulses in liquids[J]. Physical Review E, Statistical, Nonlinear, and Soft Matter Physics, 2012, 86(3): 036304.
[50] Tinne N, Knoop G, Kallweit N, et al. Effects of cavitation bubble interaction with temporally separated fs-laser pulses[J]. Journal of Biomedical Optics, 2014, 19(4): 048001.
[51] Chayen N E, Saridakis E, Sear R P. Experiment and theory for heterogeneous nucleation of protein crystals in a porous medium[J]. Proceedings of the National Academy of Sciences of the United States of America, 2006, 103(3): 597-601.
[52] Wohlgemuth K, Kordylla A, Ruether F, et al. Experimental study of the effect of bubbles on nucleation during batch cooling crystallization[J]. Chemical Engineering Science, 2009, 64(19): 4155-4163.
[53] Yuyama K, Sugiyama T, Masuhara H. Laser trapping and crystallization dynamics of l-phenylalanine at solution surface[J]. The Journal of Physical Chemistry Letters, 2013, 4(15): 2436-2440.
[54] Tu J R, Yuyama K I, Masuhara H, et al. Dynamics and mechanism of laser trapping-induced crystal growth of hen egg white lysozyme[J]. Crystal Growth & Design, 2015, 15(10): 4760-4767.
[55] Tominaga Y, Maruyama M, Yoshimura M, et al. Promotion of protein crystal growth by actively switching crystal growth mode via femtosecond laser ablation[J]. Nature Photonics, 2016, 10(11): 723-726.
[56] Suzuki D, Nakabayashi S, Yoshikawa H Y. Control of organic crystal shape by femtosecond laser ablation[J]. Crystal Growth & Design, 2018, 18(9): 4829-4833.
[57] Murakami A, Kitano H, Adachi H, et al. Universal processing technique for protein crystals using pulsed UV laser[J]. Japanese Journal of Applied Physics, 2004, 43: L873-L876.
[58] Kashii M, Kitano H, Hosokawa Y, et al. Femtosecond laser processing of protein crystals in crystallization drop[J]. Japanese Journal of Applied Physics, 2005, 44: L873-L875.
[59] Kashii M, Hosokawa Y, Kitano H, et al. Femtosecond laser-induced cleaving of protein crystal in water solution[J]. Applied Surface Science, 2007, 253(15): 6447-6450.
[60] Hasenaka H, Sugiyama S, Hirose M, et al. Femtosecond laser processing of protein crystals grown in agarose gel[J]. Journal of Crystal Growth, 2009, 312(1): 73-78.
[61] Yoshikawa H Y, Hosokawa Y, Murai R, et al. Spatially precise, soft microseeding of single protein crystals by femtosecond laser ablation[J]. Crystal Growth & Design, 2012, 12(9): 4334-4339.
[62] Wu C S, Hsieh P Y, Yuyama KI, et al. Pseudopolymorph control of l-phenylalanine achieved by laser trapping[J]. Crystal Growth & Design, 2018, 18(9): 5417-5425.
[63] Suzuki Y, Sazaki G, Matsumoto M, et al. First direct observation of elementary steps on the surfaces of glucose isomerase crystals under high pressure[J]. Crystal Growth & Design, 2009, 9(10): 4289-4295.
[64] Zhang S, Zeng X. Matthews D T A, et al. Selection of micro-fabrication techniques on stainless steel sheet for skin friction[J]. Friction, 2016, 4(2): 89-104.
[65] 龙江游, 范培迅, 龚鼎为, 等. 超快激光制备具有特殊浸润性的仿生表面[J]. 中国激光, 2016, 43(8): 0800001.
[66] Lao Z X, Hu Y L, Zhang C C, et al. Capillary force driven self-assembly of anisotropic hierarchical structures prepared by femtosecond laser 3D printing and their applications in crystallizing microparticles[J]. ACS Nano, 2015, 9(12): 12060-12069.
[67] Yoo J H, Kwon H J, Paeng D, et al. Facile fabrication of a superhydrophobic cage by laser direct writing for site-specific colloidal self-assembled photonic crystal[J]. Nanotechnology, 2016, 27(14): 145604.
[68] 孙思明, 孙允陆, 刘东旭, 等. 飞秒激光直写制备蛋白质功能化器件[J]. 激光与光电子学进展, 2013, 50(8): 080003.
[69] Applegate M B, Coburn J, Partlow B P, et al. Laser-based three-dimensional multiscale micropatterning of biocompatible hydrogels for customized tissue engineering scaffolds[J]. Proceedings of the National Acadamy of Sciences of the United States of America, 2015, 112(39): 12052-12057.
[70] Yu J C, Jiang L, Yan J F, et al. Microprocessing on single protein crystals using femtosecond pulse laser[J]. ACS Biomaterials Science & Engineering, 2020, 6(11): 6445-6452.
[71] Gamaly E G, Rode A V, Luther-Davies B, et al. Ablation of solids by femtosecond lasers: ablation mechanism and ablation thresholds for metals and dielectrics[J]. Physics of Plasmas, 2002, 9(3): 949-957.
[72] Sidhu M S, Kumar B, Singh K P. The processing and heterostructuring of silk with light[J]. Nature Materials, 2017, 16(9): 938-945.
[73] Oujja M, Pérez S, Fadeeva E, et al. Three dimensional microstructuring of biopolymers by femtosecond laser irradiation[J]. Applied Physics Letters, 2009, 95(26): 263703.
[74] Gattass R R, Mazur E. Femtosecond laser micromachining in transparent materials[J]. Nature Photonics, 2008, 2(4): 219-225.
[75] Lazare S, Sionkowska A, Zaborowicz M, et al. Bombyx mori silk protein films microprocessing with a nanosecond ultraviolet laser and a femtosecond laser workstation: theory and experiments[J]. Applied Physics A, 2012, 106(1): 67-77.
[76] Lin C Y, Li P K, Cheng L C, et al. High-throughput multiphoton-induced three-dimensional ablation and imaging for biotissues[J]. Biomedical Optics Express, 2015, 6(2): 491-499.
[77] Kerse C, Kalaycıoğlu H, Elahi P, et al. Ablation-cooled material removal with ultrafast bursts of pulses[J]. Nature, 2016, 537(7618): 84-88.
[78] Jiang L, Tsai H L. Plasma modeling for ultrashort pulse laser ablation of dielectrics[J]. Journal of Applied Physics, 2006, 100(2): 023116.
[79] Cheow W S, Xu R, Hadinoto K. Towards sustainability: new approaches to nano-drug preparation[J]. Current Pharmaceutical Design, 2013, 19(35): 6229-6245.
[80] Lee T, Kim S U, Min J H, et al. Multilevel biomemory device consisting of recombinant azurin/cytochrome C[J]. Advanced Materials, 2010, 22(4): 510-514.
[81] Torculas M, Medina J, Xue W, et al. Protein-based bioelectronics[J]. ACS Biomaterials Science & Engineering, 2016, 2(8): 1211-1223.
[82] Bostick C D, Mukhopadhyay S, Pecht I, et al. Protein bioelectronics: a review of what we do and do not know[J]. Reports on Progress in Physics, 2018, 81(2): 026601.
[83] Romanov V, Davidoff S N, Miles A R, et al. A critical comparison of protein microarray fabrication technologies[J]. The Analyst, 2014, 139(6): 1303-1326.
[84] Liu W D, Li Y F, Yang B. Fabrication and applications of the protein patterns[J]. Science China Chemistry, 2013, 56(8): 1087-1100.
[85] Launiere C, Gaskill M, Czaplewski G, et al. Channel surface patterning of alternating biomimetic protein combinations for enhanced microfluidic tumor cell isolation[J]. Analytical Chemistry, 2012, 84(9): 4022-4028.
[86] Okano K, Matsui A, Maezawa Y, et al. In situ laser micropatterning of proteins for dynamically arranging living cells[J]. Lab on a Chip, 2013, 13(20): 4078-4086.
[87] Scott M A. Wissner-Gross Z D, Yanik M F. Ultra-rapid laser protein micropatterning: screening for directed polarization of single neurons[J]. Lab Chip, 2012, 12(12): 2265-2276.
[88] Pesen D, Haviland D B. Modulation of cell adhesion complexes by surface protein patterns[J]. ACS Applied Materials & Interfaces, 2009, 1(3): 543-548.
[89] Qiao M, Wang H, Lu H, et al. Micro/nano processing of natural silk fibers with near-field enhanced ultrafast laser[J]. Science China Materials, 2020, 63: 1300-1309.
[90] Sun Y L, Sun S M, Wang P, et al. Customization of protein single nanowires for optical biosensing[J]. Small, 2015, 11(24): 2869-2876.
[91] Sun Y L, Dong W F, Niu L G, et al. Protein-based soft micro-optics fabricated by femtosecond laser direct writing[J]. Light: Science & Applications, 2014, 3(1): e129.
[92] Dinca V, Farsari M, Kafetzopoulos D, et al. Patterning parameters for biomolecules microarrays constructed with nanosecond and femtosecond UV lasers[J]. Thin Solid Films, 2008, 516(18): 6504-6511.
[93] Wu P K, Ringeisen B R, Krizman D B, et al. Laser transfer of biomaterials: matrix-assisted pulsed laser evaporation (MAPLE) and MAPLE direct write[J]. Review of Scientific Instruments, 2003, 74(4): 2546-2557.
[94] Hosokawa Y, Matsumura S, Masuhara H, et al. Laser trapping and patterning of protein microcrystals: toward highly integrated protein microarrays[J]. Journal of Applied Physics, 2004, 96(5): 2945-2948.
[95] 史志勇, 周立强, 张立春, 等. 基于多焦点阵列的动态激光并行加工[J]. 光学学报, 2020, 40(10): 1014004.
Article Outline
俞嘉晨, 闫剑锋, 李欣, 曲良体. 超快激光调控晶体形核与生长过程研究进展[J]. 中国激光, 2021, 48(2): 0202020. Jiachen Yu, Jianfeng Yan, Xin Li, Liangti Qu. Progress in Ultrafast Laser-Induced Nucleation and Crystal Growth[J]. Chinese Journal of Lasers, 2021, 48(2): 0202020.