Development of a 100 J, 10 Hz laser for compression experiments at the High Energy Density instrument at the European XFEL
1 Introduction
One of the main research goals of the High Energy Density (HED) instrument at the European X-ray Free Electron Laser (XFEL) is to study the structural and melting behaviour of materials present at the interior of extrasolar planets [ 1 ] . To support this, a 1 kW (100 J, 10 Hz, 1030 nm) nanosecond pulsed diode-pumped solid-state laser (DPSSL) developed at the Central Laser Facility (CLF) will be delivered to the European XFEL in Hamburg, Germany as a UK-funded contribution in kind to the facility. The laser, named DiPOLE100X, will provide temporally shaped pulses, which, once frequency doubled to 515 nm and focused, will be used to undertake dynamic shock and ramp compression experiments to produce extreme states of matter, replicating the high pressure (1 TPa) and temperature (up to 10,000 K) conditions found at planetary centres. Properties of key planetary materials (phase stabilities and equations of state) will then be determined by probing using high-brightness X-ray pulses from the FEL.
DiPOLE100X is the second 100 J, 10 Hz DPSSL system designed and built by the CLF and is based on a master oscillator power amplifier (MOPA) design with multiple amplification stages. It consists of a front end, operating at 1029.5 nm with gain media at room temperature, followed by two DiPOLE multi-slab ceramic Yb:YAG cryogenic gas cooled amplifiers, a 10 J cryo-preamplifier (MA1) and a final 100 J cryo-power amplifier (MA2). Figure
The DiPOLE cryogenic multi-slab amplifier concept has been developed at the CLF over the past eight years. The ceramic Yb:YAG slabs are held in aerodynamically shaped vanes and heat is removed by flowing pressurized helium gas, cooled to near cryogenic temperatures (typically around 150 K), over the slab surfaces at high velocity, a technique first demonstrated on the Mercury laser at room temperature [ 2 ] . Cryogenic cooling minimizes reabsorption loss in the Yb-system, enabling high gain and efficient energy extraction, and further improves the thermo-mechanical and thermo-optical properties of YAG, important for high-average power operation [ 3 ] . The amplifier is pumped from both sides by 940 nm diode sources with square flat-top intensity profiles to ensure a uniform gain profile. Slabs closer to the centre of the amplifier have a higher Yb-doping level to ensure a uniform thermal load in each slab, and an absorptive chromium-doped YAG cladding is added to the edge of each slab to suppress amplified spontaneous emission (ASE). Amplifier designs have been developed that produce 10 J [ 4 ] and 100 J [ 5 ] output at 10 Hz demonstrating the scalability of the concept.
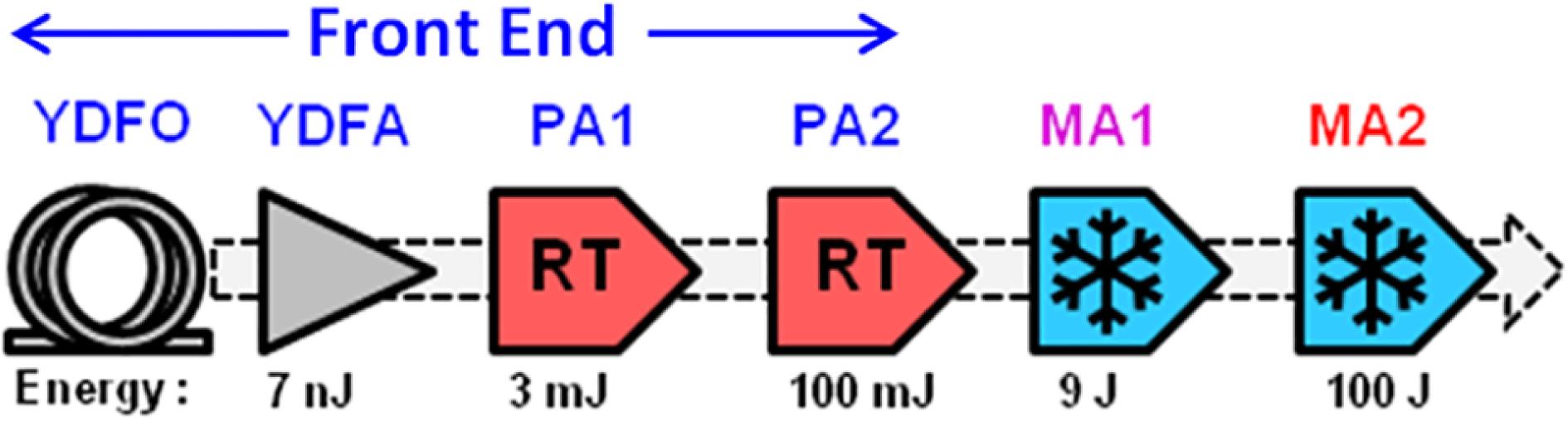
Fig. 1. Schematic of DiPOLE100X amplifier chain, showing typical output energy at each amplifier stage: YDFO
Yb–silica fibre oscillator; YDFA
Yb–silica fibre amplifier (inc. temporal pulse shaping); PA
room-temperature preamplifier (1
Yb:CaF
2
regenerative, 2
Yb:YAG multi-pass); MA
main cryogenic amplifier (ceramic Yb:YAG multi-slab).
The design of DiPOLE100X is similar to the DiPOLE100 system, the world’s first kW average power, high energy, nanosecond pulsed DPSSL [ 6 ] , which is now operational at the HiLASE facility in the Czech Republic [ 7 ] , where it is being used by a growing community of scientific and industrial users for advanced materials processing and testing applications.
The main target parameters of DiPOLE100X are summarized in Table
Table 1. Target parameters for DiPOLE100X and demonstrated performance
|
In this paper we present a progress update on the build of DiPOLE100X, highlighting design features introduced to meet the specific demands of conducting compression experiments on the HED instrument, integration of DiPOLE100X with HED infrastructure, and plans for future development of DiPOLE amplifier technology within the Centre for Advanced Laser Technologies and Applications (CALTA) at the CLF.
2 Design & build
The main difference between DiPOLE100X and its predecessor is the change from a linear to U-shape folded geometry. This was necessary to reduce the footprint and fit the shape of the space available in the laser hutch at the HED instrument, and has been achieved by reorganizing and changing the direction of propagation of the beam transport (BT) section between the 10 J and 100 J cryo-amplifiers. A 3D model showing the geometry of DiPOLE100X and highlighting some of the key components of the system is given in Figure

Fig. 2. 3D model of DiPOLE100X: FFE
fibre front end, DP
diode pumps, cGC
cryogenic gas coolers, DM
deformable mirrors, BD
beam diverter, FFE
fibre front end (not shown).
2.3 Front end
The design of the front end for DiPOLE100X is very similar to that developed for DiPOLE100
[
8
]
and a schematic of the optical arrangement, highlighting key functional areas, is shown in Figure
45 dB. This is then driven by a high sample rate (8 GSamples/s) arbitrary waveform generator (AWG, Kentech POPS100D) that provides 125 ps temporal resolution (200 ps rise time). All FFE components are contained in a single 6U 19-inch rack unit, which, along with the AWG, is located remotely from the front end optical table and connected via a fibre optic link.
A key component of the front end is the timing system that distributes pulses throughout the system, which need to be synchronized both internally and to the XFEL timing system. Accurate pulse timing and low jitter are particularly important to ensure reproducible synchronization with the X-ray pulse bursts. Figure
To achieve accurate synchronization, DiPOLE100X has an upgraded 40-channel digital delay generator (DDG – Greenfield Technology GFT1040) that accepts two external input signals from the XFEL timing system; an 81.25 MHz signal clock derived from the XFEL master oscillator, and a 10 Hz (
) trigger signal synchronized with the start of each X-ray burst
[
9
]
. Using the clock-in the DDG generates a 40.625 MHz clock signal (PA1 synchronization) and a clock-out to synchronize the delay generator of the FFE. The external 10 Hz trigger is used directly (frequency
) to generate a user selectable frequency at 5, 2, or 1 Hz (
). Every channel of the DDG produces one pulse with adjustable delay at one of these two frequencies. Finally, the XFEL timing system provides a 10 kHz trigger directly to the FFE delay generator to minimize jitter between the shaped optical pulse and X-ray pulse. The jitter between individual channels and the
XFEL trigger is expected to be
20 ps (RMS). A timing diagram for DiPOLE100X is shown in Figure

Fig. 6. Schematic showing 7-pass angularly multiplexed extraction architecture of the 10 J cryo-preamplifier. DP
diode pumps, DM1
10 J deformable mirror, BS
beam splitters.
The temporally shaped output of the FFE at 10 kHz is used to seed the Yb:CaF 2 regenerative amplifier (PA1) (custom S-PULSE supplied by Amplitude Systemes, France) producing few-mJ pulses at 10 Hz in a Gaussian spatial beam profile. The cavity length in PA1 has been increased to support the increased pulse duration range required for DiPOLE100X.
The beam is then spatially shaped into a square high-order super-Gaussian (
) beam and relay-imaged into the Yb:YAG multi-pass amplifier (Lastronics, Germany) boosting the energy beyond 100 mJ. The output then passes through a Pockels cell to improve contrast and to pick pulses at the desired output pulse rate of the laser, if this is set to less than 10 Hz.
10 J cryo-preamplifier
The 10 J multi-pass cryo-preamplifier (MA1) design in DiPOLE100X also remains unchanged from the original. It consists of a single DiPOLE10-scale cryo-amplifier head and a seven pass angularly multiplexed extraction architecture
[
5
]
. A schematic of the optical arrangement showing the extraction architecture is given in Figure
In DiPOLE100X the original deformable mirror (DM), positioned after the 3rd pass, has been replaced with a similar low-voltage bimorph-type mirror developed and built at the CLF
[
10
]
. The DM uses a 75 mm diameter fused silica substrate, approximately 1 mm thick, bonded to a 50 mm diameter piezo-ceramic (PZT) disc, approximately
thick. Both surfaces of the fused silica substrate are coated with a high reflectivity dielectric mirror coating at 1030 nm for use at normal incidence. A photograph of the new 10 J bimorph mirror is shown in Figure
square array similar in size to the 10 J beam (21.5 mm square), see inset of Figure
The mirror was tested on the DiPOLE prototype amplifier system, described elsewhere
[
4
]
, and demonstrated closed-loop correction with an output wave front error (WFE) of
peak to valley (PV) and 31 nm (RMS) when amplifying 10 ns duration pulses to 10 J at 10 Hz. An image of the corrected output wave front measured on the DiPOLE prototype amplifier is shown in Figure

Fig. 7. (a) Photograph of the DiPOLE100X 10 J bimorph deformable mirror, built at the CLF, with inset showing schematic of electrode pattern, (b) corrected output wave front and (c) far-field CCD camera image measured at 10 J, 10 Hz on the DiPOLE prototype amplifier.

Fig. 8. Energy stability over half an hour with inset showing measured temporal pulse shape for amplification of 2.2 ns pulses at 8 J, 10 Hz.
The prototype amplifier was also used to confirm reliable operation at the shorter pulse durations required for DiPOLE100X that are of interest for shock compression experiments. Output energy stability tests were undertaken for amplification of a near flat-top temporal pulse of duration 2.2 ns (FWHM) at 10 Hz, and demonstrated 8 J output with an RMS energy stability of 0.5% over half an hour operation (21,600 shots). Figure
) used during these tests. This gives confidence that DiPOLE100X will be able to deliver the necessary performance over the extended range of pulse durations required for HED experiments.
The output from the 10 J cryo-preamplifier passes through a Faraday isolator before entering the beam transport section of the system. Here the polarization state and spatial orientation of the beam can be adjusted to precompensate for effects that occur in the final cryo-amplifier. The beam is then relay-imaged into a
3.4 magnification telescope to increase its size to 75 mm square ready to seed the main 100 J amplifier. The magnifying telescope is now orientated at right angles to the original design to achieve the new U-shaped layout.
100 J cryo-power amplifier
The final cryo-amplifier in DiPOLE100X also retains the same architecture used in DiPOLE100. Both diode pumps (Ingeneric, Germany) are directed into the amplifier on-axis and a 4-pass, off-axis, angularly multiplexed geometry is used to extract the stored energy
[
5
]
. A schematic showing the extraction architecture of the 100 J cryo-amplifier is given in Figure

Fig. 9. Schematic showing 4-pass, off-axis, angularly multiplexed extraction architecture of the 100 J cryo-amplifier. DP
diode pumps, DM2
100 J deformable mirror, BD
beam diverter.
The main change in the system is the incorporation of an upgraded DM between the first and second amplifier passes. The new DM (Imagine Optic ILAO Star 200) is based on upgraded mechanical actuator technology driven by stepper motors providing nanometric precision, now with very low hysteresis (
1%). These result in minimal backlash allowing real-time dynamic closed-loop wave front correction at up to 10 Hz. The circular mirror substrate is 200 mm in diameter and its shape is controlled by an array of 52 actuators. The mirror is mounted in a new low-profile, low distortion optical mount, a photograph of which is shown in Figure
Prior to installation on DiPOLE100X the mirror was tested off-line as part of a closed-loop adaptive optic (AO) system, including a HASO4 Broadband-GE Shack–Hartmann wave front sensor (WFS). To confirm the mirror is able to provide smooth dynamic correction to the level required a beam of similar size and shape to the 100 J beam with an initially flat wave front was incident on the mirror. The DM was then programmed to create a wave front consistent with the level of aberration expected in the 100 J amplifier. The target wave front is shown in Figure

Fig. 10. (a) Photograph of new 100 J deformable mirror, (b) target aberrated wave front and (c) residual error in generated wave front.
After closed-loop operation the target wave front was successfully recreated with a residual error of
(PV) and approximately 40 nm (RMS). A map of the residual error measured is shown in Figure
Whenever the output beam is not sent to the HED interaction area, during warm-up and testing, it is reflected off a beam diverter (BD) and absorbed in a water filled beam dump. The diverter consists of a low mass high reflectivity dielectric coated mirror mounted and attached to a linear guide providing up to 160 mm of travel. A low friction, position-encoded linear motor (Linmot, Switzerland) provides precise movement (speed and acceleration) of the mirror assembly. The diverter allows the mirror to be lowered out of, or raised into, the beam, in less than 100 ms. Movement of the diverter is synchronized to the triggering of the laser to ensure a laser pulse is not present when the mirror is travelling between positions. When the diverter mirror is in the lower position, the beam propagates through to a periscope, which in turn directs the beam downwards through the optical table into the high energy beam transport system; see Section
The diverter is also used to provide the user with a single shot on demand pulse picking capability. In this mode, the front end output Pockels cell and both 10 J and 100 J cryo-amplifiers are operated at 1 Hz to maintain stable thermal conditions. When the user requests a pulse the mirror is moved out of the beam before the next available pulse arrives, and is then returned to its original position after the pulse has passed.
2.6 Control system
Control of the many components and sub-systems that make up the DiPOLE100X laser is provided by a distributed control system developed at the CLF using EPICS (Experimental Physics and Industrial Control System) [ 11 ] . EPICS is an open source software framework, providing a collection of tools and applications for use in creating distributed control systems, which is used in many large-scale science facilities, such as particle accelerators and synchrotron light sources.
The control system is run from central Linux-based servers. Distributed access and control are provided by a graphical user interface (GUI), developed using Control System Studio (CSS) software
[
12
]
, which may be installed on any Windows-based PC connected to the system network. The user interface of the control system is broken down into a set of control screens whose functions are described in Table
A separate programmable logic controller (PLC) provides local system monitoring and control, simultaneously providing local machine safety, sequencing components, and periodically publishing process variables (PVs) to EPICS, such that the master system is aware of each sub-systems status. A separate archiving toolset is included in EPICS that automatically records requested PVs as a function of time and stores them to disk for later retrieval.
Personnel safety is paramount, and a separate personnel safety system monitors the condition and operation of a number of interlocks. These provide a means of shutting the system down in an emergency situation through connection to the laboratory safety system. The control and safety systems continuously perform checks to prevent operation unless all safety criteria are met.
Table 2. Functionality of user control screens in DiPOLE100X control system
|

Fig. 11. Synoptic screen for control and monitoring of 10 J cryo-preamplifier. Red lines correspond to the main 1030 nm laser beam path, input from the FE (left) and output to the beam transport section (right); blue lines represent diagnostic beam paths; and orange lines correspond to 940 nm pump diode beam paths.
2.7 Temporal pulse shaping
Shock and ramp compression experiments require precise control of laser pulse shape. To achieve this DiPOLE100X will incorporate a dynamic closed-loop temporal pulse shaping capability to account for the various nonlinear factors (saturation characteristics) that affect the pulse as it passes through the amplifier chain. The approach adopted uses an initial empirical estimate of the seed pulse shape, which is applied to the FEOM in the FFE via the AWG. The generated optical pulse is then amplified by the laser chain and its shape detected at the output by the combination of a high bandwidth fibre-coupled InGaAs PIN photo detector (CIM Model ISIS PHB-AJ-24E, rise & fall time
70 ps, cut-off frequency
5 GHz) and digital storage oscilloscope (Rhode & Schwarz RTO2044, 4 GHz, 4-channel, 20 GSa/s per channel). The output pulse shape is then compared to the target shape in software and a correction calculated by a custom pulse shaping algorithm developed in-house. The corrected pulse shape is then reapplied to the FEOM by the AWG. The process is then repeated over a series of iterations until the target pulse shape is reached within defined acceptance criteria.
The accuracy of shape reproduction is dependent on several factors, including the stability, resolution, and bandwidth of the AWG and FEOM, the energy stability of the laser chain, and the noise and jitter of the detection and signal acquisition system. The pulse contrast achievable is limited by the dynamic range of the FEOM and residual ASE. To control the speed at which the pulse shape converges to the target solution, and minimize the risk of optical damage due to rapid pulse shape changes, a damping factor is included in the calculation with the aim of reaching the target shape within five to ten iterations when operated at maximum pulse rate (10 Hz).
Prior to commissioning on DiPOLE100X, the DiPOLE prototype amplifier has been used to test the pulse shaping software and optimize the algorithm in a representative amplifier chain. Results of two tests undertaken at an output energy of 6.5 J at 10 Hz are shown in Figure

Fig. 12. Temporal pulse shaping results at 6.5 J, 10 Hz obtained using the DiPOLE prototype amplifier (a) flat-top and (b) multi-step pyramid pulse profiles.
These results demonstrate the temporal pulse shaping capability expected from DiPOLE100X and confirm the potential to undertake dynamic single shock (flat top, high temperature, ramp low temperature) and multiple-shock (step pulse) compression experiments on the HED instrument.
2.8 Build status
Build of DiPOLE100X at the CLF commenced in March 2017 with installation of the optical tables in the laboratory. Installation of front end components was completed in May 2017 with first pulsed operation demonstrated in June 2017. The main 10 J sub-systems (pump diodes, amplifier head and cryo-cooling system) were installed at the end of September 2017 followed by all opto-mechanical components by March 2018. Full alignment of the 10 J was completed in May 2018 and pulsed commissioning is now underway. All the main 100 J sub-systems were installed by January 2018 and the majority of opto-mechanical components by June 2018. Alignment of the 100 J is scheduled to commence in July 2018 with pulsed commissioning of the full system beginning in August 2018. Control system integration and machine safety testing have occurred throughout the build with final commissioning occurring in parallel with final pulsed operation tests. At the time of writing, the build is expected to be completed by the end of September 2018.
A collection of time lapse photographs showing build progression over the past 15 months is shown in Figure

Fig. 13. Time lapse photographs of DiPOLE100X build with 3D CAD view of completed system.
3 Integration at HED instrument
The HED instrument at the European XFEL is arranged with the laser bay, or hutch, on a raised floor above the interaction area where the X-ray probe beam from the SASE II beamline enters. A schematic of the main components of the HED instrument is shown in Figure
The laser hutch contains the two main lasers; the high energy (HE) nanosecond pulsed laser, DiPOLE100X that will be used for dynamic compression experiments, and a commercial high intensity (HI) laser from Amplitude Technologies (
300 TW, 25 fs, Ti:S), which will be used to study relativistic plasmas. DiPOLE100X occupies approximately half the floor area of the laser hutch with ancillary control racks, chillers and the 100 J cryostat located in a separate plant room. An HE beam transport system relay-images the output beam from DiPOLE100X beneath the floor of the laser hutch and down to an optical table alongside the interaction chamber. There it will be frequency doubled to 515 nm before being focused using phase plates into the interaction chamber to achieve the desired flat-top focal intensity profile to conduct experiments. Figure
4 Technology development
In parallel with DiPOLE100X development, separate projects within CALTA are looking at extending the performance of DiPOLE technology, demonstrating its potential for advanced materials processing applications
[
13
]
, and developing a compact pump source technology for ultra-high intensity petawatt-class lasers. These offer the potential to generate compact radiation (X-ray,
-ray) and particle (electron, proton etc.) sources with applications in nondestructive testing, high resolution radiography
[
14
]
, and the next generation of laser plasma-based accelerators
[
15
]
.
DiPOLE technology development falls into four main research activities. The first extends the pulse repetition rate of DiPOLE from 10 Hz up to 100 Hz; the second scales the energy of the existing DiPOLE100 design towards 150 J; the third is targeted at demonstrating efficient and stable frequency conversion of DiPOLE systems into the visible (SHG at 515 nm) and UV (third harmonic generation at 343 nm), under high pulse energy and high-average power conditions; and the final activity is looking at reducing the size of DiPOLE systems.
To achieve these aims a prototype 10 J, 100 Hz DiPOLE laser is currently under development at the CLF, initially focused on industrial materials processing applications. To enable energy scaling of DiPOLE100 technology within the same design envelope work is underway to improve the damage resilience of key optical components (gain media, mirrors etc.). Furthermore, long term stability trials of SHG and THG under high-average power conditions are underway to provide extended capability for materials processing and large beam area LIDT testing, and to demonstrate the potential of DiPOLE as a pump source technology. These activities are undertaken in collaboration with the HiLASE Facility with funding support from the Czech Republic and the European Commission [ 16 ] . A separate internally funded project is investigating design options for size reduction of key DiPOLE component technologies (such as cryo-coolers) important for the realization of practical applications.
5 Summary
DiPOLE100X is a high energy DPSSL laser designed to produce 100 J, temporally shaped nanosecond duration pulses at up to 10 Hz in a compact geometry based on the original DiPOLE100 design. At the time of writing, all the major opto-mechanical components have been installed and commissioning of the cryo-amplifiers has begun. Testing at the CLF is scheduled for completion in autumn 2018 before shipment to the European XFEL by the end of the year.
The DiPOLE prototype amplifier has been used to confirm operation at shorter pulse durations, and the ability of DiPOLE100X to provide precisely programmed temporal pulses at up to 10 Hz repetition rate will allow determination of optimum laser pulse shapes for shock compression experiments in real time. This capability at the HED instrument, coupled with high-brightness X-ray probe pulses from the XFEL beamline, will revolutionize our understanding of the structure of materials and the processes that occur in extreme high energy density environments.
[1] 1.M.McMahon and U.Zastrau ,Conceptual Design Report – Dynamic Laser Compression Experiments at the HED Instrument of European XFEL , XFEL.EU TR-2017-001, February 2017.
[8] 8.T.Butcher ,P. D.Mason ,S.Banerjee ,J.Pilar ,M.Divoky ,J.Smith ,M.De Vido ,J.Phillips ,K.Ertel ,O.Chekhlov ,S.Tomlinson ,W.Shaikh ,J.Greenhalgh ,I.Musgrave ,C.Hernandez-Gomez , and J.Collier , in Advanced Solid State Lasers ( OSA Technical Digest , 2014 ), paper ATh2A.50.
[9] 9.G.Priebe ,U.Zastrau , and M.Lederer ,Timing Interfacing between XFEL and HIBEF User Consortium , XFEL.EU 21-04-2016-3, April 2016.
[10] 10.C. J.Hooker ,J. L.Collier ,S. J.Hawkes , and C.Spindloe ,Central Laser Facility Annual Report 2005/6 , (2006), p. 202.
[12] 12.http://controlsystemstudio.org/ .
[13] 13.J.Nygaard ,The Laser User86 , 22 ( 2017 ).
[15] 15.P. A.Walker ,P. D.Alesini ,A. S.Alexandrova ,M. P.Anania ,N. E.Andreev ,I.Andriyash ,A.Aschikhin ,R. W.Assmann ,T.Audet ,A.Bacci ,I. F.Barna ,A.Beaton ,A.Beck ,A.Beluze ,A.Bernhard ,S.Bielawski ,F. G.Bisesto ,J.Boedewadt ,F.Brandi ,O.Bringer ,R.Brinkmann ,E.Bründermann ,M.Büscher ,M.Bussmann ,G. C.Bussolino ,A.Chance ,J. C.Chanteloup ,M.Chen ,E.Chiadroni ,A.Cianchi ,J.Clarke ,J.Cole ,M. E.Couprie ,M.Croia ,B.Cros ,J.Dale ,G.Dattoli ,N.Delerue ,O.Delferriere ,P.Delinikolas ,J.Dias ,U.Dorda ,K.Ertel ,A.Ferran Pousa ,M.Ferrario ,F.Filippi ,J.Fils ,R.Fiorito ,R. A.Fonseca ,M.Galimberti ,A.Gallo ,D.Garzella ,P.Gastinel ,D.Giove ,A.Giribono ,L. A.Gizzi ,F. J.Grüner ,A. F.Habib ,L. C.Haefner ,T.Heinemann ,B.Hidding ,B. J.Holzer ,S. M.Hooker ,T.Hosokai ,A.Irman ,D. A.Jaroszynski ,S.Jaster-Merz ,C.Joshi ,M. C.Kaluza ,M.Kando ,O. S.Karger ,S.Karsch ,E.Khazanov ,D.Khikhlukha ,A.Knetsch ,D.Kocon ,P.Koester ,O.Kononenko ,G.Korn ,I.Kostyukov ,L.Labate ,C.Lechner ,W. P.Leemans ,A.Lehrach ,F. Y.Li ,X.Li ,V.Libov ,A.Lifschitz ,V.Litvinenko ,W.Lu ,A. R.Maier ,V.Malka ,G. G.Manahan ,S. P. D.Mangles ,B.Marchetti ,A.Marocchino ,A.Martinez de la Ossa ,J. L.Martins ,F.Massimo ,F.Mathieu ,G.Maynard ,T. J.Mehrling ,A. Y.Molodozhentsev ,A.Mosnier ,A.Mostacci ,A. S.Mueller ,Z.Najmudin ,P. A. P.Nghiem ,F.Nguyen ,P.Niknejadi ,J.Osterhoff ,D.Papadopoulos ,B.Patrizi ,R.Pattathil ,V.Petrillo ,M. A.Pocsai ,K.Poder ,R.Pompili ,L.Pribyl ,D.Pugacheva ,S.Romeo ,A. R.Rossi ,E.Roussel ,A. A.Sahai ,P.Scherkl ,U.Schramm ,C. B.Schroeder ,J.Schwindling ,J.Scifo ,L.Serafini ,Z. M.Sheng ,L. O.Silva ,T.Silva ,C.Simon ,U.Sinha ,A.Specka ,M. J. V.Streeter ,E. N.Svystun ,D.Symes ,C.Szwaj ,G.Tauscher ,A. G. R.Thomas ,N.Thompson ,G.Toci ,P.Tomassini ,C.Vaccarezza ,M.Vannini ,J. M.Vieira ,F.Villa ,C.-G.Wahlström ,R.Walczak ,M. K.Weikum ,C. P.Welsch ,C.Wiemann ,J.Wolfenden ,G.Xia ,M.Yabashi ,L.Yu ,J.Zhu , and A.Zigler ,J. Phys. Conf. Ser.874 , 012029 ( 2017 ).
[16] 16.Supported by the state budget of the Czech Republic (projects LO 1602, LM2015086).
Article Outline
Paul Mason, Saumyabrata Banerjee, Jodie Smith, Thomas Butcher, Jonathan Phillips, Hauke Höppner, Dominik Möller, Klaus Ertel, Mariastefania De Vido, Ian Hollingham, Andrew Norton, Stephanie Tomlinson, Tinesimba Zata, Jorge Suarez Merchan, Chris Hooker, Mike Tyldesley, Toma Toncian, Cristina Hernandez-Gomez, Chris Edwards, John Collier. Development of a 100 J, 10 Hz laser for compression experiments at the High Energy Density instrument at the European XFEL[J]. High Power Laser Science and Engineering, 2018, 6(4): 04000e65.