张应变Ge1-xSnx合金导带结构调控
下载: 754次
1 引言
硅基光源问题是制约硅基光电集成技术进一步发展的瓶颈。Ge1-xSnx合金通过调节Sn组分可实现从间接带隙到直接带隙材料的转变,同时随着高质量Ge1-xSnx材料的成功生长[1-4],科学家们提出了利用与硅工艺相兼容的Ge1-xSnx合金来实现高效发光的新思路。自2015年Wirths等[2]首次报道了边发射光泵激光器以来,Ge1-xSnx直接带隙发光材料的外延与器件的制备等工作获得了极大的发展,代表性成果包括2016年报道的2.5 μm硅基Ge1-xSnx边发射光泵激光器[3]、微盘光泵激光器[5],2017年报道的波长范围为2~3 μm的光泵激光器[6],以及2019年报道的电注入型垂直腔面发射发光器件[7]。由于Sn在Ge中的固溶度低等缺点,生长高组分、高质量的Ge1-xSnx材料较困难;目前制备得到的激光器普遍存在非电注入、工作温度低等缺点,有待进一步从理论、材料和器件设计等方面进行优化。
在Ge1-xSnx能带计算方面,各研究小组采用的研究方法主要可归纳为两类。第一类是采用第一性原理方法进行计算。例如:文献[ 8]通过计算发现,由于Sn原子的电负性较弱,Sn原子向Ge原子的电荷转移很小,导致当Sn的组分(物质的量分数)约为3.1%时,Ge1-xSnx合金可被调控为直接带隙材料。然而文献[ 9]计算得到Sn的组分约为6.5%时,才能将Ge1-xSnx合金调控为直接带隙材料。文献[ 10]计算了双轴(100)、(110)、(111)晶面应变Ge1-xSnx能带结构转变为直接带隙结构的条件,结果表明Sn的组分约为8.5%时弛豫的Ge1-xSnx可以被调控为直接带隙材料。由于文献[ 10]没有考虑应变条件下导带的退简并作用,计算得到在(100)、(110)晶面的张应变和(111)晶面的压应变作用下,可通过减少Sn的组分使之转换成直接带隙材料。综上所述,Ge1-xSnx合金带隙转换条件还存在争议。
第二类是采用基于实验参数的半经验方法,如紧束缚近似、k·p理论、形变势理论等[11-14]。文献[ 15]用30带k·p方法研究了无应变Ge1-xSnx能带,得到能带参数随Sn的组分的变化关系,并预测Sn的组分约为7.3%时Ge1-xSnx合金可转化为直接带隙材料。与基于第一性原理的方法相比,该方法具有计算速度快,计算值更接近于实验结果,可灵活移植至器件仿真模型,方便提供关键参数及优化设计等优点。
一方面,目前鲜有将单轴应变Ge1-xSnx调控为直接带隙材料的理论计算;另一方面,可以通过SiN应力薄膜技术[16-17]、微盘结构设计[18]等方法,在Ge1-xSnx有源层中引入张应变。基于此,本文采用形变势理论,研究张应变Ge1-xSnx合金在不同类型应变条件下的导带结构调控。首先,建立Ge1-xSnx在(001)、(110)、(111)晶面的双轴以及[001]、[110]、[111]晶向的单轴应变张量模型;其次,以Ge0.97Sn0.03 为例计算导带直接带隙和间接带隙L能谷的带边在不同类型应变条件下的变化趋势;最后,综合考虑应变和组分共同作用下的张应变Ge1-xSnx导带结构调控规律,并得出可将Ge1-xSnx调控为直接带隙材料的应变和组分的函数关系式。
2 理论建模
2.1 应变张量模型
根据胡克定律以及坐标变换方法可得,应变张量可以写成含有6个独立变量的二阶对称张量[19],即
式中:εxx、εyy、εzz分别表示沿x、y、z主轴方向的线应变;εxy、εyz、εzx分别表示沿主轴方向旋转的剪切应变,且有εij=εji。对Ge1-xSnx 的(001)、(110)、(111)3个典型晶面施加双轴应变,则对应的应变张量可表示为
式中:
式中:
式中:
对Ge1-xSnx的[001]、[110]、[111]3个典型晶向施加单轴应变,则对应的应变张量可表示为
式中:
式中:
式中:
2.2 带隙调控模型
有了应变张量模型,根据形变势理论[20-22]就可以得到Ge1-xSnx导带中不同类型的能谷(ki)相对于应变的变化关系,即
式中:
考虑到弯曲系数会对Ge1-xSnx带隙产生重要影响,本研究选择通过30带k·p方法及实验数据拟合得到的结果作为弛豫的Ge1-xSnx导带直接带和间接带能谷的带隙值,即[15]
Ge1-xSnx导带含有3个能谷(Γ、X和L),且三重简并间接带隙的X能谷位置更高,虽然剪切应变分量也能使其退简并,但是在张应变条件下它对将Ge1-xSnx带隙调控为直接带隙的影响不大,因此,本研究只给出直接带隙Γ和间接带隙L能谷随应变的转换关系;在计算过程中也忽略了应变对价带的影响。
表 1. Ge与Sn材料的相关参数[10,13,15]
Table 1. Parameters for Ge and Sn[10,13,15]
|
3 结果与分析
3.1 应变张量分析
对于(110)面双轴张应变,εzz>εxx=εyy,如
在[001]晶向单轴张应变的作用下,轴向应力沿着z轴,因此εzz正比于平面内应变ε⊥,同时应变分量因泊松效应有εxx=εyy<0,如
对于[110]晶向单轴张应变,剪切应变εxy>εxx=εyy,将使得L能谷发生退简并;同时εzz<0,εyz=εxz=0,如
![平面内应变与各应变分量的关系。(a) (001)面双轴张应变;(b) (110)面双轴张应变;(c) (111)面双轴张应变;(d) [001]晶向单轴张应变;(e) [110]晶向单轴张应变;(f) [111]晶向单轴张应变](/richHtml/lop/2020/57/9/091602/img_1.jpg)
图 1. 平面内应变与各应变分量的关系。(a) (001)面双轴张应变;(b) (110)面双轴张应变;(c) (111)面双轴张应变;(d) [001]晶向单轴张应变;(e) [110]晶向单轴张应变;(f) [111]晶向单轴张应变
Fig. 1. Various strain components as a function of in-plane strain. (a) Biaxial tensile strain paralleled to the (001) plane; (b) biaxial tensile strain paralleled to the (110) plane; (c) biaxial tensile strain paralleled to the (111) plane; (d) uniaxial tensile strain paralleled to the [001] direction; (e) uniaxial tensile strain paralleled to the [110] direction; (f) uniaxial tensile strain paralleled to the [111] direction
3.2 双轴张应变Ge1-xSnx合金带隙调控
为了便于直观分析比较Ge1-xSnx合金的带隙随应变的变化规律,以Ge0.97Sn0.03为例,分别计算了(001)、(110)、(111)晶面双轴张应变下其导带直接带隙Γ和间接带隙L能谷位置的偏移情况,结果如

图 2. 不同类型双轴张应变条件下Ge0.97Sn0.03带隙与应变的关系。(a) (001)面双轴张应变;(b) (110)面双轴张应变;(c) (111)面双轴张应变
Fig. 2. Changes in the bandgaps of Ge0.97Sn0.03 with various type of strains. (a) Biaxial tensile strain paralleled to the (001) plane; (b) biaxial tensile strain paralleled to the (110) plane; (c) biaxial tensile strain paralleled to the (111) plane
对于(110) Ge0.97Sn0.03材料,双轴张应变使得导带直接带隙Γ能谷以每增加1%的应变则下降151.91 meV的速度减小;由于剪切应变分量εxy不为零,则四重简并的间接带隙L能谷分裂成两个二重简并的子带(记为L1,4和L2,3)。L1,4随着应变的增加而增大,增大的速度为37.19 meV/%,并与直接带隙Γ能谷相交;L2,3能谷随着张应变的增加而减小,减小的速度为106.74 meV/%,直接带隙Γ能谷的下降速度是该速度的1.42倍,这意味着(110)面双轴张应变调控Ge0.97Sn0.03为直接带隙将的难度比(001)面大,所需的张应变约为2.0%。
对于(111) Ge0.97Sn0.03材料,双轴张应变使得导带直接带隙Γ能谷以每增加1%的应变则下降159.86 meV的速度减小;同样由于剪切应变分量εxy不等于零,则四重简并的间接带隙L能谷分裂成一个非简并的L1子带和一个三重简并的子带(记为L2,3,4)。其中L1随着应变的增加而增大,并与直接带隙相交。而子带L2,3,4随着张应变的增加而减小,减小的速度为172.66 meV/%,该速度是直接带隙Γ能谷下降速度的1.08倍,这意味着(111)面张应变的Ge0.97Sn0.03不能调控为直接带隙材料。
为了研究张应变和Sn的组分共同作用对Ge1-xSnx带隙调控的影响,将直接带隙Γ与间接带隙L能谷之间的差值绘成二维等能图,如
计算结果表明,若外延Ge1-xSnx材料的Sn的组分较低(x<0.082),可以通过诸如施加SiN应力层及微盘结构设计[18]等方法来提高Ge1-xSnx层的(001)或(110)面双轴张应变,从而将其调控为直接带隙材料;但此策略对于(111)面无效。
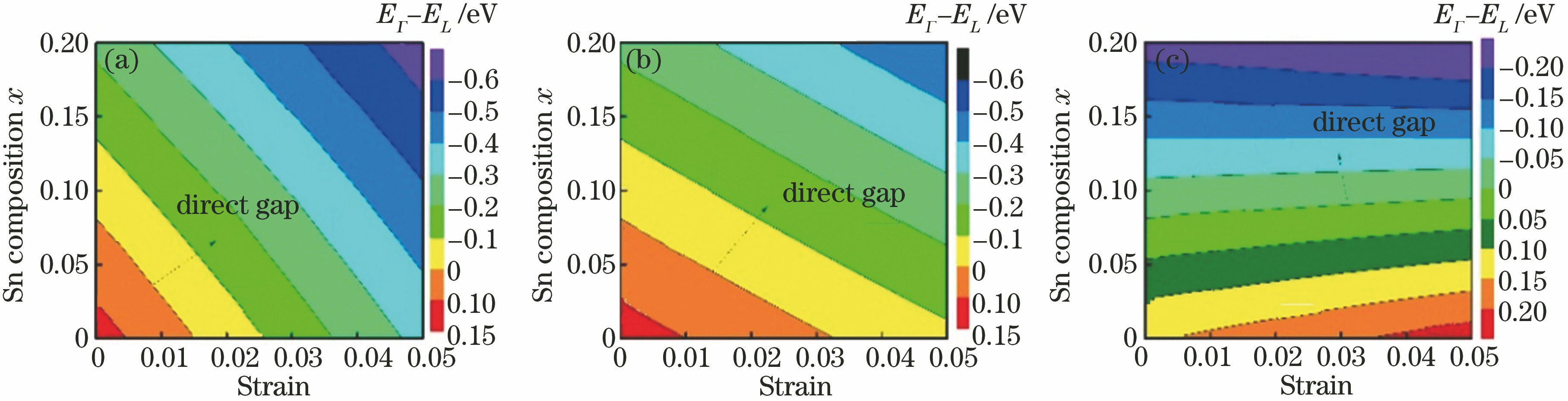
图 3. 不同类型双轴张应变条件下Ge1-xSnx带隙调控情况。(a)(001)面双轴张应变;(b) (110)面双轴张应变;(c) (111)面双轴张应变
Fig. 3. Energy separation between the direct and indirect conduction band minima in Ge1-xSnx as a function of strain and Sn composition with various type of strains. (a) Biaxial tensile strain paralleled to the (001) plane; (b) biaxial tensile strain paralleled to the (110) plane; (c) biaxial tensile strain paralleled to the (111) plane
由
3.3 单轴张应变Ge1-xSnx合金带隙调控
以Ge0.97Sn0.03 为例,分别计算了[001]、[110]、[111]晶向单轴张应变下其导带直接带隙Γ和间接带隙L能谷位置的偏移情况,如
对于[110] Ge0.97Sn0.03材料,单轴张应变使得导带直接带隙Γ能谷以每增加1%的应变则下降24.31 meV的速度减小;四重简并的间接带隙L能谷分裂成两个二重简并的子带。其中L1,4随着张应变的增加而增大,增大的速度为61.96 meV/%,并与直接带隙Γ能谷相交。而L2,3能谷随着张应变的增加而减小,减小的速度为73.09 meV/%,该速度远大于直接带隙Γ能谷的下降速度,因此[110]晶向单轴张应变条件下不能将Ge0.97Sn0.03调控为直接带隙材料。
同理,将[111]单轴张应变施加于Ge0.97Sn0.03材料上,虽然剪切应变分量的存在使得L能谷发生退简并,但由于导带直接带隙Γ能谷的下降速度(46.56 meV/%)略小于间接带隙L能谷的下降速度(52.01 meV/%),因此[111]晶向单轴张应变不能将Ge0.97Sn0.03调控为直接带隙材料。
![不同类型单轴张应变条件下Ge0.97Sn0.03带隙与应变的关系。(a) [001]晶向单轴张应变;(b) [110]晶向单轴张应变;(c) [111]晶向单轴张应变](/richHtml/lop/2020/57/9/091602/img_4.jpg)
图 4. 不同类型单轴张应变条件下Ge0.97Sn0.03带隙与应变的关系。(a) [001]晶向单轴张应变;(b) [110]晶向单轴张应变;(c) [111]晶向单轴张应变
Fig. 4. Changes in the bandgaps of Ge0.97Sn0.03 with various type of strains. (a) Uniaxial tensile strain paralleled to the [001] direction; (b) uniaxial tensile strain paralleled to the [110] direction; (c) uniaxial tensile strain paralleled to the [111] direction
![不同类型单轴张应变条件下Ge1-xSnx带隙调控情况。(a)[001]晶向单轴张应变;(b) [110]晶向单轴张应变;(c) [111]晶向单轴张应变](/richHtml/lop/2020/57/9/091602/img_5.jpg)
图 5. 不同类型单轴张应变条件下Ge1-xSnx带隙调控情况。(a)[001]晶向单轴张应变;(b) [110]晶向单轴张应变;(c) [111]晶向单轴张应变
Fig. 5. Energy separation between the direct and indirect conduction band minima in Ge1-xSnx as a function of strain and Sn composition with various type of strains. (a) Uniaxial tensile strain paralleled to the [001] direction; (b) uniaxial tensile strain paralleled to the [110] direction; (c) uniaxial tensile strain paralleled to the [111] direction
由于目前实验条件的限制,不同类型张应变条件下Ge1-xSnx的制备难度较大,已有研究中几乎没有相关报道。为了进一步说明本研究计算的正确性,将计算得到Ge材料在不同类型张应变条件下被调控为直接带隙材料所需要施加的应变值与文献[
23-25]的计算结果进行比较,如
表 2. 将Ge材料调控为直接带隙材料所需要的张应变值
Table 2. Critical strains for Ge under biaxial and uniaxial tension strain where the indirect-to-direct band-gap transition occurs
|
4 结论
基于形变势理论研究了(001)、(110)、(111)晶面双轴张应变以及[001]、[110]、[111]晶向单轴张应变Ge1-xSnx导带结构。采用所提方法计算得到的将弛豫型Ge1-xSnx调控为直接带隙材料所需的Sn的组分约为8.2%。结果表明,不同类型的张应变施加于Ge1-xSnx合金时,直接带隙Γ能谷和间接带隙L能谷下降的速度存在竞争关系。在张应变为[0%,5%],Sn的组分范围为[0,0.2],(001)面双轴张应变条件下Ge1-xSnx具有最大的带隙调控面积,其次为(110) 面双轴张应变,第三为[001]晶向单轴张应变,[110]晶向单轴张应变的带隙调控面积最小。可以通过对Ge1-xSnx施加(001)、(110)晶面双轴张应变以及[001]晶向轴张应变的方法来减小Ge1-xSnx合金中的Sn的组分,从而获得直接带隙材料。本研究进一步完善了将Ge1-xSnx合金调控为直接带隙材料方法的相关理论,可为Ge1-xSnx材料生长、器件仿真及制备等提供关键参数和理论指导。
[1] 苏少坚, 张东亮, 张广泽, 等. Ge(001) 衬底上分子束外延生长高质量的Ge1-xSnx合金[J]. 物理学报, 2013, 62(5): 058101.
Su S J, Zhang D L, Zhang G Z, et al. High-quality Ge1-xSnx alloys grown on Ge(001) substrates by molecular beam epitaxy[J]. Acta Physica Sinica, 2013, 62(5): 058101.
[2] Wirths S. Geiger R, von den Driesch N, et al. Lasing in direct-bandgap GeSn alloy grown on Si[J]. Nature Photonics, 2015, 9(2): 88-92.
[3] Al-Kabi S, Ghetmiri S A, Margetis J, et al. An optically pumped 2.5 μm GeSn laser on Si operating at 110 K[J]. Applied Physics Letters, 2016, 109(17): 171105.
[4] Liu Z, Cong H, Yang F, et al. Defect-free high Sn-content GeSn on insulator grown by rapid melting growth[J]. Scientific Reports, 2016, 6: 38386.
[5] Stange D, Wirths S, Geiger R, et al. Optically pumped GeSn microdisk lasers on Si[J]. ACS Photonics, 2016, 3(7): 1279-1285.
[6] Margetis J, Al-Kabi S, Du W, et al. Si-based GeSn lasers with wavelength coverage of 2-3 μm and operating temperatures up to 180 K[J]. ACS Photonics, 2018, 5(3): 827-833.
[7] Huang B J, Chang C Y, Hsieh Y D, et al. Electrically injected GeSn vertical-cavity surface emitters on silicon-on-insulator platforms[J]. ACS Photonics, 2019, 6(8): 1931-1938.
[8] Wang X H, Chen C Z, Feng S Q, et al. A hybrid functional first-principles study on the band structure of non-strained Ge1-xSnx alloys[J]. Chinese Physics B, 2017, 26(12): 127402.
[9] Polak M P, Scharoch P, Kudrawiec R. The electronic band structure of Ge1-xSnx in the full composition range: indirect, direct, and inverted gaps regimes, band offsets, and the Burstein-Moss effect[J]. Journal of Physics D: Applied Physics, 2017, 50(19): 195103.
[10] Huang W Q, Cheng B W, Xue C L, et al. Comparative studies of band structures for biaxial (100)-, (110)-, and (111)-strained GeSn: a first-principles calculation with GGA+U approach[J]. Journal of Applied Physics, 2015, 118(16): 165704.
[11] Gupta S, Magyari-Köpe B, Nishi Y, et al. Achieving direct band gap in germanium through integration of Sn alloying and external strain[J]. Journal of Applied Physics, 2013, 113(7): 073707.
[12] Liu S Q, Yen S T. Extraction of eight-band k·p parameters from empirical pseudopotentials for GeSn[J]. Journal of Applied Physics, 2019, 125(24): 245701.
[13] Attiaoui A, Moutanabbir O. Indirect-to-direct band gap transition in relaxed and strained Ge1-x-ySixSny ternary alloys[J]. Journal of Applied Physics, 2014, 116(6): 063712.
[14] Moontragoon P. Ikoni Z, Harrison P. Band structure calculations of Si-Ge-Sn alloys: achieving direct band gap materials[J]. Semiconductor Science and Technology, 2007, 22(7): 742-748.
[15] Song Z G, Fan W J, Tan C S, et al. Band structure of Ge1-xSnx alloy: a full-zone 30-band k · p model[J]. New Journal of Physics, 2019, 21(7): 073037.
[16] Zhang Q F, Liu Y, Han G Q, et al. Theoretical analysis of performance enhancement in GeSn/SiGeSn light-emitting diode enabled by Si3N4 liner stressor technique[J]. Applied Optics, 2016, 55(34): 9668-9674.
[17] Liu Y, Fang C Z, Gao X, et al. Theoretical investigation of tensile-strained GeSn/SiGeSn multiple quantum well laser wrapped in Si3N4 liner stressor[J]. IEEE Photonics Journal, 2018, 10(1): 1500609.
[18] Millar R W. Dumas D C S, Gallacher K F, et al. Mid-infrared light emission > 3 μm wavelength from tensile strained GeSn microdisks[J]. Optics Express, 2017, 25(21): 25374-25385.
[19] Yoo K H, Albrecht J D. Ram-Mohan L R. Strain in layered zinc blende and wurtzite semiconductor structures grown along arbitrary crystallographic directions[J]. American Journal of Physics, 2010, 78(6): 589-597.
[20] Martin R M. Theoretical calculations of heterojunction discontinuities in the Si/Ge system[J]. Physical Review B, 1986, 34(8): 5621-5634.
[21] . Band lineups and deformation potentials in the model-solid theory[J]. Physical Review B, 1989, 39(3): 1871-1883.
[22] Fischetti M V, Laux S E. Band structure, deformation potentials, and carrier mobility in strained Si, Ge, and SiGe alloys[J]. Journal of Applied Physics, 1996, 80(4): 2234-2252.
[23] Tahini H, Chroneos A, Grimes R W, et al. Strain-induced changes to the electronic structure of germanium[J]. Journal of Physics: Condensed Matter, 2012, 24(19): 195802.
[24] Inaoka T, Furukawa T, Toma R, et al. Tensile-strain effect of inducing the indirect-to-direct band-gap transition and reducing the band-gap energy of Ge[J]. Journal of Applied Physics, 2015, 118(10): 105704.
[25] Liu L, Zhang M, Hu L J, et al. Effect of tensile strain on the electronic structure of Ge: a first-principles calculation[J]. Journal of Applied Physics, 2014, 116(11): 113105.
Article Outline
孙钦钦, 黄诗浩. 张应变Ge1-xSnx合金导带结构调控[J]. 激光与光电子学进展, 2020, 57(9): 091602. Qinqin Sun, Shihao Huang. Calculation of Conduction Band Structure Tensile Strained Ge1-xSnx Alloys for Achieving Direct Band Gap Materials[J]. Laser & Optoelectronics Progress, 2020, 57(9): 091602.