Plasmonic resonance-linewidth shrinkage to boost biosensing
Download: 705次
1. INTRODUCTION
Surface plasmon resonance (SPR) [1,2] with tunable resonance wavelength, linewidth, and intensity has proven instrumental in wide applications, such as physics [3–
In order to improve SPR sensing performances, substantial efforts have been devoted to increasing the FOM of plasmonic sensors [18
On the one hand, reduction of non-radiative losses can efficiently prolong , generally focusing on improving the crystallinity of metallic nanomaterials and seeking alternatives with low losses [20,31], such as dielectric materials. On the other hand, reduction of radiative losses can be achieved through the coupling of plasmonic modes associated with localized surface plasmon (LSP) and surface plasmon polaritons (SPPs) [15,18,3234" target="_self" style="display: inline;">–
In this work, we systematically investigate the plasmonic effects on biomolecular sensing by changing excitation conditions, such as the incident angle, azimuthal angle, and polarization. We reveal the abrupt shrinking of resonance linewidth in the direction, which is attributed to the hybridized mode induced by the interference of two degenerate SPP modes. In view of the unique resonance feature, we adopt the hybridized mode to demonstrate the practical biosensing capability by detecting the prostate specific antigen (PSA), which is a key biomarker for the diagnosis and prognosis of prostatic cancer. By optimizing the excitation conditions, a maximum sensitivity is achieved in the direction, and the manipulation on light polarization and angle demonstrates significant improvement on biosensing performance.
2. RESULTS AND DISCUSSION
2.1 A. Plasmonic Resonance-Linewidth Shrinking
Two-dimensional (2D) gold nanohole arrays are fabricated via the nanoimprint lithography system and the gold deposition method [43]. The detailed experimental procedure is depicted in the Materials and Methods section. The schematic illustration of the measurement configuration is shown in Fig.
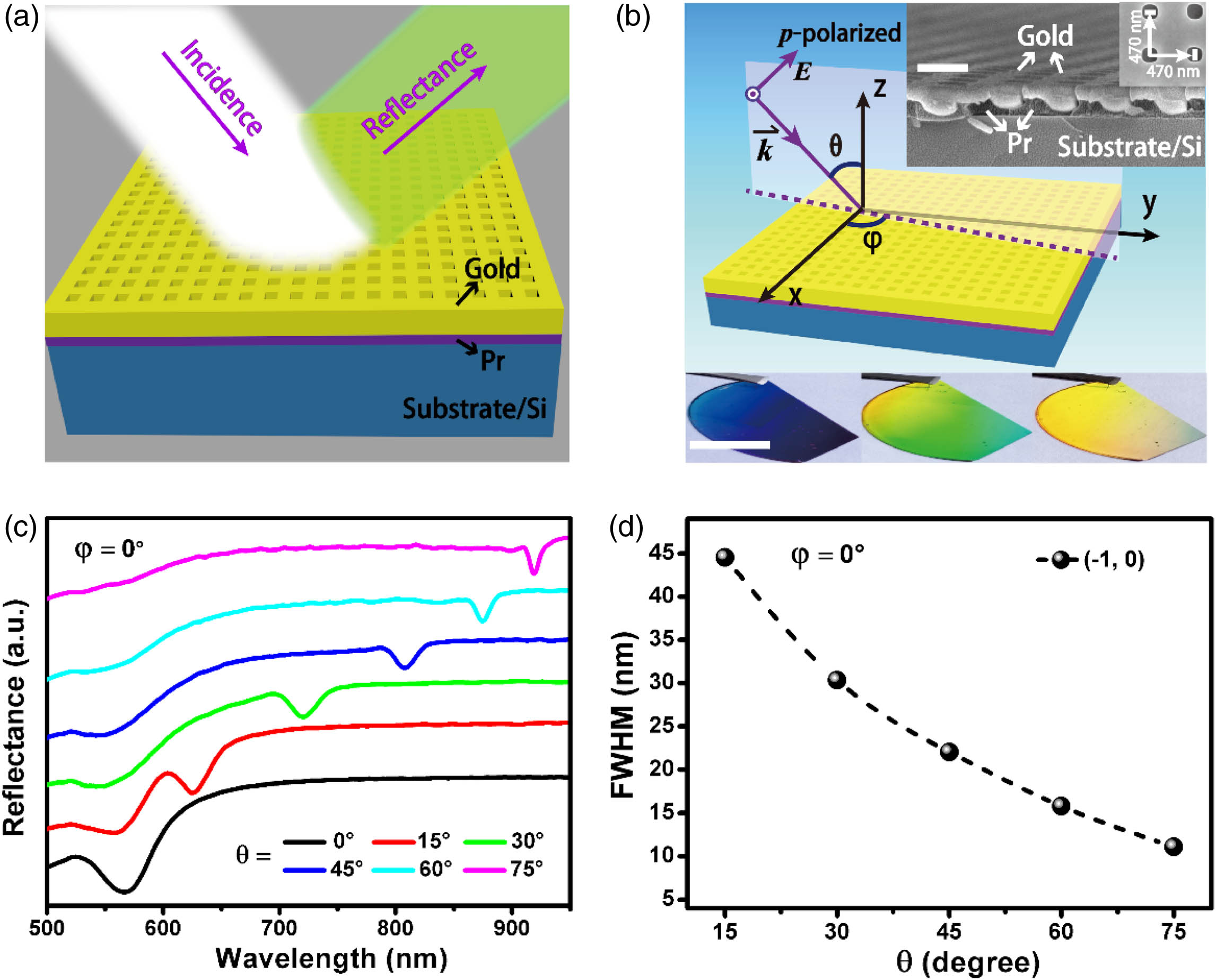
Fig. 1. Two-dimensional gold nanohole arrays supporting SPP modes fabricated by the nanoimprint lithography method. (a) Schematic illustration of the optical measurement configuration. (b) Definitions of incident angle , azimuthal angle , and polarization ( or ), respectively. Inset: (top) SEM images of the array with cross-sectional and top views; (bottom) photographic images of the array under different visual angles. Scale bars denote 500 nm and 1 cm for SEM and photographic images, respectively. (c) Experimentally measured reflectance spectra as a function of incident angles from 0° to 75°. The azimuthal angle is set as . (d) Dependence of FWHM on ( , 0) SPP mode achieved at different incident angles.
The gold nanohole arrays provide orthogonal reciprocal lattice vectors, allowing for exciting grating-coupled SPP modes and trapping spatial light around resonance wavelengths. In comparison with the off-resonance modes (see Appendix
In order to find out how the azimuthal angle influences resonance wavelengths and linewidths of plasmonic modes, we study the reflectance spectra depending on the change of azimuthal angle. As illustrated in Fig.

Fig. 2. Tuning the linewidths of plasmonic modes by varying azimuthal angles. (a), (b) Azimuthal angle-dependent reflectance spectra at the incident angles of 15° and 75°, respectively. (c) Theoretical resonance wavelength of ( , 0) mode (black curve) and (0, ) (red curve) mode as a function of the azimuthal angle. Measured ( , 0) mode (black spheres) and (0, ) mode (red spheres) nearly overlaid on theoretical modes. The pink dash arrows denote the cases used later. (d) Dependence of FWHM on ( , 0) mode (blue spheres) and (0, ) mode (red spheres) achieved at different azimuthal angles. The short dash line and dot correspond to the left and right coordinate values, respectively, as shown by black arrows. Th incident angle is set as in both (c) and (d).
2.2 B. Efficient Biomolecular Sensing
Based on above investigations of SPR linewidths and intensities, we have demonstrated that the FWHM decreases with increasing and that an abrupt change of the FWHM occurs at . The plasmonic modes with narrow FWHM are highly advantageous for ultrasensitive SPR sensing, and are particularly beneficial for biomolecular detection [9,10,47]. Therefore, we have exemplified the sensing of PSA by the immunologic specificity of anti-PSA binding. For reference, the whole detailed experimental procedures of functionalization, detection, and recycling have been depicted in our previous work [9], as briefly illustrated in Appendix
To systematically validate and demonstrate the influences of excitation conditions on the biosensing performance, we have measured the detection sensitivity and FOM of the array under different excitation configurations. As illustrated in Fig.
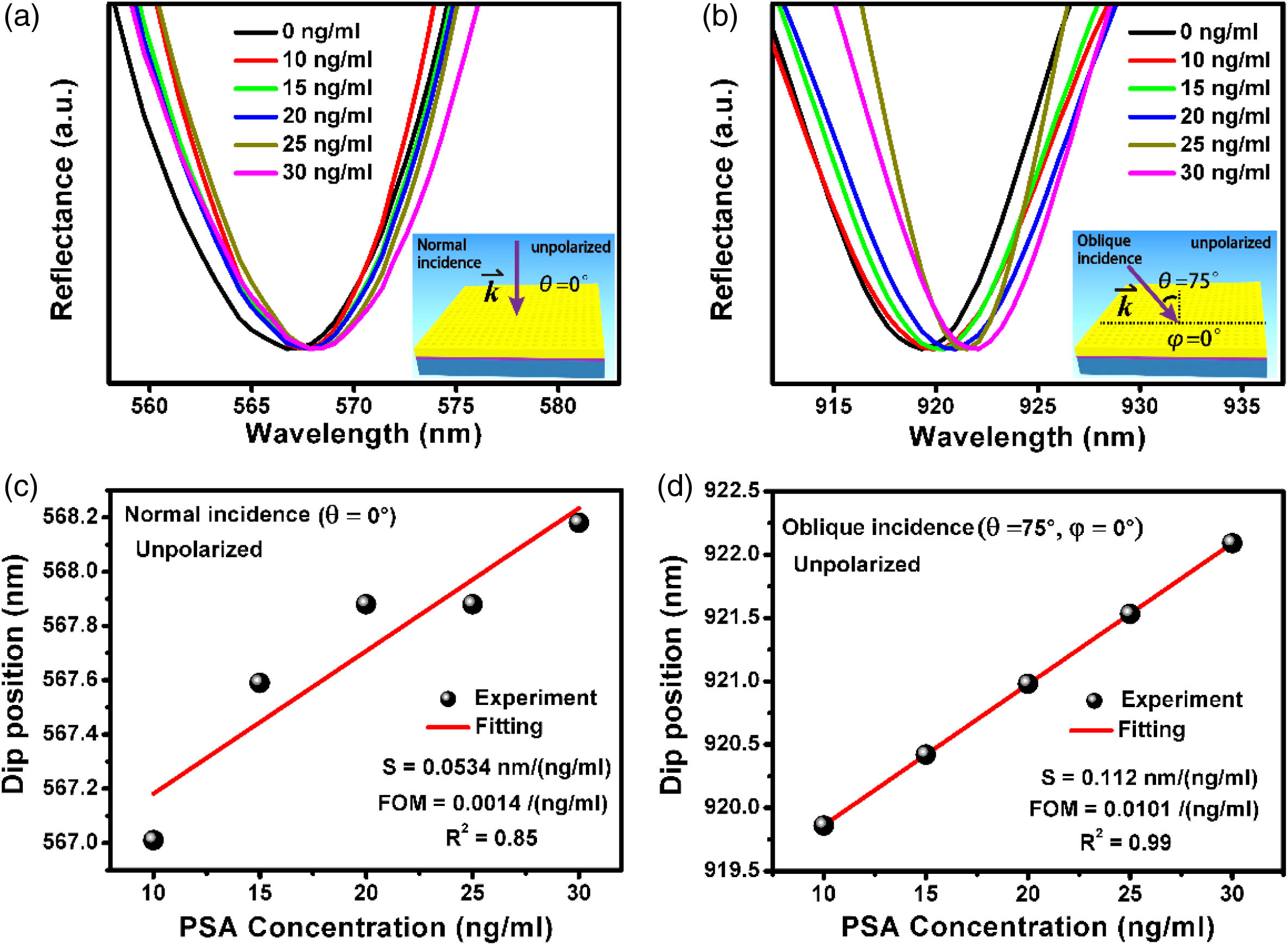
Fig. 3. Comparisons of the sensitivity between normal and oblique incidence with the unpolarized light. (a), (b) Experimentally measured reflectance spectra with different PSA concentrations ranging from 10 to 30 ng/mL after normalization. The excitation configurations are set as normal ( ) and oblique ( ) incidence with the unpolarized light in (a) and (b), respectively. Inset: Schematic drawings of excitation configurations. (c), (d) Plots of resonance wavelength positions of plasmonic modes extracted from (a) and (b) against PSA concentrations. The and denote the sensitivity and the correlation coefficient of the linear fitting, respectively. Note that the FWHM values are about 44 nm and 11 nm in (a) and (b), respectively.
We continue to investigate the influence of the azimuthal angle on the detection sensitivity [Fig.

Fig. 4. Comparisons of the sensitivity under different azimuthal angles with the -polarized light. (a), (b) Experimentally measured reflectance spectra with different PSA concentrations ranging from 10 to 35 ng/mL after normalization. Inset: Schematic drawings of excitation configurations. (c), (d) Resonance wavelength positions of plasmonic modes extracted from (a) and (b) as a function of PSA concentration. The and denote the sensitivity and the correlation coefficient of the linear fitting, respectively. Note that the FWHM values are about 10 nm and 12 nm in (a) and (b), respectively.
Comparisons of biosensing performance under different excitation configurations are summarized in Table
Table 1. Comparisons of Biosensing Performance under Different Excitation Configurationsa
|

Fig. 5. Comparisons of the (a) sensitivity and (b) FOM under different excitation configurations. The C1, C2, C3, and C4 represent corresponding configurations explicated in Table 1 .
Additionally, this study also features several advantages for practical applications. First, plasmonic characteristics such as resonance wavelength, linewidth, and intensity can be conveniently tuned in a broadband covering the visible and near IR range. Assuming that the material is replaced to aluminum, the spectral band is capable of covering the ultraviolet range [48]. Second, the working wavelength of the optimal sensitivity lies in the visible range, which facilitates the use of low-cost detecting devices such as a charge-coupled digital camera. Third, the concentration of PSA detected in our biosensing experiment can be as low as 10 ng/mL, beyond the threshold concentration of 20 ng/mL in the early cancer prediction. Furthermore, under the excitation condition of C4, highly confined and enhanced electromagnetic fields can be formed around the corner of the nanohole [40,49], indicating the potential of our approach for single-molecule sensing [50,51].
3. CONCLUSION
In conclusion, we have systematically investigated the plasmonic effects of Au nanohole arrays through changing excitation conditions, such as the incident angle, azimuthal angle, and polarization. Importantly, an abrupt shrinking effect of resonance linewidth originated from the interference of two degenerate SPP modes in the direction has been revealed. Moreover, we have demonstrated the biosensing capability for detecting the molecular biomarker of prostatic carcinoma under different excitation conditions, and achieved the maximum sensitivity in the direction. Our study may deepen the understanding of plasmonic resonance-linewidth shrinking, and offer a promising strategy for improving biosensing performance significantly.
4. MATERIALS AND METHODS
4.1 A. Sample Fabrication
The clean silicon wafer was prepared after the standard cleaning procedure detailed previously. Then the wafer was dried and baked in an oven at 150°C for 2 h. After drying, the Si wafer was spin-coated with the photoresist (Tu-170, Obducat, Sweden) for 200 nm. Afterwards, the spin-coated wafer was processed with the nanoimprint system. Then, the chromium and gold layers were deposited sequentially with an electron-beam evaporation system (FC2000, Temescal). The depth of the hole is set as after optimization.
4.2 B. Characterizations
Normal incidence and oblique angle-resolved reflectance spectra were measured by a lab-built system, mainly including a light source (AvaLight-DH-S-BAL, Avantes), a portable UV-visible-NIR spectrometer (AvaSpec-NIR256/512-1, Avantes), and a film polarizer (LPVISE2X2, Thorlabs). The morphology of the array was characterized by scanning an electron microscope (S-4800, Hitachi).
4.3 C. Simulation
The theoretical reflection spectrum (Appendix
5 Acknowledgment
Acknowledgment. The authors would like to thank Prof. Martin Moskovits and Dr. Feng Zhao for helpful discussions.
[1] M. Moskovits. Surface-enhanced spectroscopy. Rev. Mod. Phys., 1985, 57: 783-826.
[2]
[44] C. Genet, T. W. Ebbesen. Light in tiny holes. Nature, 2007, 445: 39-46.
[52] P. B. Johnson. Optical constants of the noble metals. Phys. Rev. B, 1972, 6: 4370-4379.
[53] C. Zhao, J. Chen, H. Li, T. Li, S. Zhu. Mode division multiplexed holography by out-of-plane scattering of plasmon/guided modes. Chin. Opt. Lett., 2018, 16: 070901.
[54] F. Gan, C. Sun, H. Li, Q. Gong, J. Chen. On-chip polarization splitter based on a multimode plasmonic waveguide. Photon. Res., 2018, 6: 47-53.
Article Outline
Min Gao, Weimin Yang, Zhengying Wang, Shaowei Lin, Jinfeng Zhu, Zhilin Yang. Plasmonic resonance-linewidth shrinkage to boost biosensing[J]. Photonics Research, 2020, 8(7): 07001226.