铜微纳结构的激光直写及其应用研究进展
下载: 1322次
Significance Owing to excellent adaptability to different working conditions, flexible electronics have attracted significant attention in many fields, such as wireless communication, human-machine interaction, and personal healthcare. Functional parts and conductive circuits are the basic components of electronics that respond to external stimulus and conduct signals, respectively.
Nanomaterials with unique physical and chemical properties are widely used for developing flexible electronics. Noble metals, such as silver, gold, and platinum are good candidates for manufacturing conductive parts because of their high conductivity and chemical stability. However, the high price of these metals limits their large scale production. Recently, copper has been considered a good alternative to noble metals for developing conductive component owing to its low-cost and excellent electrical properties. Furthermore, copper oxides (cuprous oxide and cupric oxide) are important transition metal oxides because of their semiconductive properties. They have been widely used as functional parts owing to their high sensitivity for external stimulation, such as humidity, temperature. Efficient manufacturing methods for materials play a major role in developing high-performance devices.
The typical “bottom-up” process, such as hydrothermal and chemical precipitation, provides a low-cost, precise control, and large-scale synthesis route to manufacture the micro/nanostructured copper. However, post-treatment processes, such as printing and sintering, are required to obtain the desired properties in a device. Such step-by-step manufacturing requires the cooperation of various techniques, which increases the process cost and complexity. Thus, developing a low-cost process for manufacturing the micro/nanostructures has attracted significant attention.
Direct laser writing, as an advanced processing technology developed recently, provides a novel approach for micro/nanostructure manufacturing. This technology has been used to process the structure, including noble metals, metal oxides, and carbon-based materials. In this study, the technical characteristics of manufacturing copper-based micro/nanostructures with direct laser writing have been summarized.
Progress The typical laser processing of micro/nanostructures, such as laser assembly, sintering, and synthesis, has been elaborated (
Conclusions and Prospect In summary, direct laser writing has been an efficient manufacturing process for copper micro/nanostructures owing to its noncontact, maskless, and rapid processing characteristics. Direct laser writing based on ionic precursors integrates the synthesis, positioning, assembly, and joining of copper nanomaterials into a one-step, which shows unique advantages in structure and composition control. This process still faces challenges in processing copper structures, such as the accurate control of products, diversification of composite structures, and further expansion of application. Further, in-depth study is needed to explore the writing mechanism and fully understand the processing characteristics for copper-based micro/nanostructures.
1 引言
相对于传统电子,新兴的柔性电子具有更大的灵活性,能够在一定程度上适应不同的工作环境,满足设备的形变要求,在无线通信、多功能娱乐、个人医疗保健等多个方面有着广泛的应用[1-2]。典型的柔性电子系统由导电结构及功能结构组成,其中,功能结构通过可变的电性能对外部环境如应力、应变、温度、化学介质等进行响应;导电结构用于传输电力及响应信号。理想的器件可连续、稳定、密切地监测人类/机械活动而不会对其造成干扰或产生限制[3],因而对器件的材料性能有着极高要求。
具有独特物理化学性质的纳米材料在柔性电子领域应用广泛。对于导电结构,贵金属纳米材料(如金、银、铂等)因其优良的导电性及化学稳定性而备受关注[4-6]。然而,贵金属纳米材料的资源有限且成本高昂,限制了其在大规模柔性电子生产中的应用。铜(Cu)因其资源丰富(较银产能高1000倍)、成本低(约为银价格的1%)、固有导电性高(略低于银,且比金高)等优点,有望取代贵金属纳米材料,继而降低导电结构的制造成本[7]。此外,铜氧化物(氧化铜及氧化亚铜)纳米结构为重要的半导体,常作为功能结构集成到微电子器件中[8-10]。纳米材料的制造可分为“自上而下”和“自下而上”两类工艺。其中,“自下而上”工艺的材料利用率高,能精细地调控微纳结构,具有更低的制造成本[6]。在传统的“自下而上”过程中,首先基于“湿化学法”(例如水热法、化学沉淀法等)完成纳米材料的合成。通过对溶液成分、pH值、环境温度等反应条件进行调控,可以精确地控制所得纳米材料的形貌、成分及结构[11]。随后,采用图形化技术(如丝网印刷、喷墨打印等)将所得纳米材料进行组装/结构化[12]。此外,还需要后处理工艺对结构性能进一步调控(如热退火、热烧结等)。分步制造需要多种技术的配合,增加了工艺成本且过程复杂。因此,开发低成本微纳结构的制造技术是近年来研究的热门课题之一。
激光加工利用激光束与材料相互作用时产生的加热、熔化、汽化、形成等离子体、烧蚀等效应来完成[13]。作为近年飞速发展起来的先进加工技术,激光加工为微/纳米结构制造提供了新途径[14-15]。目前,激光加工已被广泛应用于各种微纳材料的制造,如贵金属、金属氧化物、碳系材料(如石墨烯和氧化石墨烯)等[13,16-20]。本文从“自下而上”的微纳结构激光加工方法入手,列举了激光组装、激光烧结/连接、激光合成及结构化在微/纳米结构制备中的应用,并与其他制造方法进行了对比。重点讨论了基于铜离子前驱体的激光直写方法,详细论述了前驱体成分/状态以及激光工艺参数对铜基结构的组织、成分、电学性能的影响。分析了激光直写铜微纳结构性能的调控方法,并针对其工艺难点、应用方向、发展趋势进行了展望。
2 “自下而上”的微纳结构激光加工
可利用激光将分散于溶液中的预合成纳米粒子在目标基板上进行组装/图形化。相较于常用的喷墨打印技术[12],非接触式激光组装/图案化避免了诸如喷头堵塞、喷头污染带来的加工系统损耗,减小了其对结构化质量的影响。如
![典型微纳结构的“自下而上”激光加工。(a)光诱导组装[21];(b)激光烧结(与炉中烧结对比)[27];(c)光诱导水热法合成[28]](/richHtml/zgjg/2021/48/8/0802012/img_1.jpg)
图 1. 典型微纳结构的“自下而上”激光加工。(a)光诱导组装[21];(b)激光烧结(与炉中烧结对比)[27];(c)光诱导水热法合成[28]
Fig. 1. Typical “bottom-up” laser processing of micro/nano structures. (a) Laser-induced assembly[21]; (b) comparison of laser sintering and bulk heating[27]; (c) laser-induced hydrothermal growth[28]
除通过分子间作用力完成纳米材料组装外,可通过激光辐照完成纳米材料的连接/烧结。传统的炉中烧结技术需要整体升温,通常需要在高温下进行(>300 ℃),不仅耗时且易造成柔性基板烧损[6]。如
激光不仅可加工预合成纳米材料,且可基于离子态前驱体完成纳米材料的合成。与水热法类似,激光合成纳米材料亦可通过氧化还原反应进行。如
3 激光直写铜基微纳结构及其电性能调控
3.1 激光直写导电铜结构工艺
基于预合成的铜纳米材料,可利用激光直写完成其连接及图形化。由于快速、低热作用的工艺特点,铜纳米材料的激光烧结无需额外的保护气氛/真空环境[38]。由于不同类型的激光具有不同的加工特性,选择合适的激光类型(尤其是激光波长)有利于抑制铜纳米材料在烧结时的氧化。例如,光诱导的SPR效应仅会在纳米结构的结节处引起局部温升形成连接,可有效避免整体加热引起的铜结构氧化。对于薄层(数纳米至数十纳米)铜纳米线的烧结而言,532 nm波长的激光(对应铜纳米线共振波长)是较优选择[27];由于激光的穿透深度与其波长正相关,对于厚层(数百纳米至微米级)纳米铜结构的烧结,近红外波长的激光更具优势:采用1064 nm波长的激光烧结铜纳米颗粒时,热量将主要向深度方向传导,仅表面与空气接触的铜纳米材料发生少量氧化[29]。但预合成的铜纳米材料容易在储存过程中氧化,导致烧结所需的能量升高,最终结构的导电性降低[39]。因此采用激光烧结铜材料前,需要额外的预处理步骤去除氧化物薄膜[40]。为克服这一问题,可采用具有更低成本、更易保存的铜氧化物作为前驱体[41]。在铜氧化物(氧化铜或氧化亚铜)中加入还原剂后,可利用激光直写将铜氧化物还原为铜纳米材料,并进一步完成其烧结。一方面,激光通过热作用分解还原剂,完成铜纳米材料的还原。另一方面,激光辐照铜氧化物引起的电子跃迁也将促进其还原[42]。激光热效应与波长正相关,但光子能量与波长成反比。光诱导电子跃迁需要光子能量高于氧化物的带隙,由于氧化铜的带隙(约1.2 eV)低于氧化亚铜(约2.1 eV)[43],故氧化铜可匹配更长波长的激光(兼具光-热作用及光致电子跃迁作用),更适合作为前驱体使用。但该还原-烧结进程仍是基于预合成材料。从工艺流程简化及成本节约的角度出发,基于离子态前驱体的激光直写一步法被认为是更加高效的铜微纳结构制造技术。离子态前驱体由铜盐及还原剂组成,激光辐照将诱导其中还原剂分解并合成铜纳米结构,同时将其原位连接为导电结构[44-45]。基于离子态前驱体的激光直写不仅可规避预合成铜纳米材料的氧化问题,且将铜纳米材料的合成、烧结及结构化整合为一个步骤,极大地提高了铜微纳结构的制造效率。
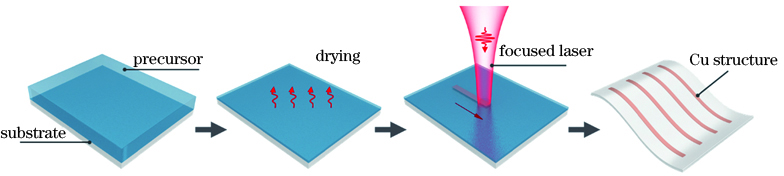
图 2. 基于离子态铜盐前驱体的激光直写典型工艺流程
Fig. 2. Typical manufacturing process of the direct laser writing based on the copper ionic precursor
还原剂是成功合成铜微纳结构的关键。具有羟基(—OH基团)的醇/多元醇,如甲醇、乙醇、异丙醇等均可用于铜纳米材料的合成[43]。其中,乙二醇因其沸点高、蒸发速率慢等特点被认为是较优的还原剂[43]。一般来讲,多元醇通过分解形成的醛基起到了还原作用[51]:
含羧基(—COOH基团)的有机物及无机物[例如聚乙烯吡咯烷酮(PVP)、乙酸(CH3COOH)、甲酸(HCOOH)]也可用于铜纳米材料的还原[49]。以PVP为例,激光热作用将PVP分解为亚甲基结构、甲胺和丙酸。丙酸将进一步分解成甲酸并将Cu2+还原为C
值得注意的是,激光诱导的还原/合成过程很大程度上取决于氧化还原的反应温度。这意味着虽然激光可将升温区域局限在非常小的区域内,但仍然需要达到对应的温度阈值。例如多元醇的分解温度在180 ℃左右[43],而甲酸约为100 ℃[53]。由于含羧基还原剂的分解温度更低,含羧基还原剂将比多元醇更适用于在热敏基板上直写导电铜结构。
激光工艺参数是影响直写结构特征尺寸、成分及微观组织的重要因素。激光的光斑直径决定了直写铜结构的线宽。由于大多激光的光束能量为高斯分布,其中心区域的热输入要高于边缘[19,48]。如
![激光工艺参数对直写铜结构的影响[48]。(a)线宽;(b)成分;(c)微观组织](/richHtml/zgjg/2021/48/8/0802012/img_3.jpg)
图 3. 激光工艺参数对直写铜结构的影响[48]。(a)线宽;(b)成分;(c)微观组织
Fig. 3. Effect of laser parameters on the written copper structure[48]. (a) Width of structure; (b) composition;(c) microstructure
3.2 激光直写铜基微纳结构的电性能及其调控
对于铜导电结构而言,导电性是其性能评价的重要指标之一。在获得高铜含量的导电结构后,其导电性能主要受到孔隙率的影响。
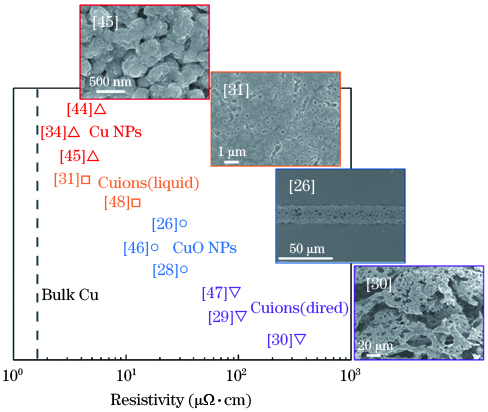
图 4. 基于不同前驱体直写所得典型铜微结构的电阻率及微观形貌比较
Fig. 4. Comparison of resistivity and microstructure of the laser written copper structures based on different precursors
对于导电铜微纳米结构而言,其氧化问题不仅存在于储存及加工过程中,服役过程中结构的氧化也将导致其导电性能损失[59]。在完成导电铜结构的制备后,通常利用封装层[如聚二甲基硅氧烷(PDMS)[44]、聚甲基丙烯酸甲酯(PMMA)[60]等]来隔绝空气,避免结构氧化。显然额外的封装工艺将使导电结构的制造过程复杂化,提高了成本。制备铜基核壳结构(如铜@银[61-63]、铜@金-银合金[64]、铜@镍[65]、铜@石墨烯[66]等)是提高结构稳定性的有效手段。通过对激光直写所用前驱体成分的调控,可完成复合结构的制备。如
![激光直写铜基复合微纳结构。(a)铜碳复合结构的形成机理示意[45];(b)典型铜碳复合结构的微观形貌及元素分布;(c)铜碳复合结构与铜结构的抗氧化性能比较[47];(d)低激光功率下直写铜氧化物的结构[48]](/richHtml/zgjg/2021/48/8/0802012/img_5.jpg)
图 5. 激光直写铜基复合微纳结构。(a)铜碳复合结构的形成机理示意[45];(b)典型铜碳复合结构的微观形貌及元素分布;(c)铜碳复合结构与铜结构的抗氧化性能比较[47];(d)低激光功率下直写铜氧化物的结构[48]
Fig. 5. Direct laser writing of Cu-based composite micro-nano structure. (a) Schematic diagram of the formation mechanism of the Cu-C composite structure[45]; (b) microstructure and elements distribution of the typical Cu-C composite structure; (c) comparison of oxidation resistance of Cu and Cu-C structures[47]; (d) structure of the directly written Cu oxides at a low laser power[48]
此外,虽对于导电铜基结构而言,氧化物是不期望的缺陷,但对于功能结构而言,铜氧化物具有半导体的特点,具有开发为功能结构的潜力。激光直写为功能结构及导电结构的可控制备提供了新思路。如
4 直写铜微纳结构的典型应用
4.1 导电铜结构的应用
导电电极是激光直写铜微纳结构的典型应用之一。如
![导电铜结构的典型应用。(a)柔性电极[47];(b)应变传感器对手指运动的监测[44];(c)接近/触摸传感器对手指触摸的响应[71];(d)柔性平面天线及其频宽[45]](/richHtml/zgjg/2021/48/8/0802012/img_6.jpg)
图 6. 导电铜结构的典型应用。(a)柔性电极[47];(b)应变传感器对手指运动的监测[44];(c)接近/触摸传感器对手指触摸的响应[71];(d)柔性平面天线及其频宽[45]
Fig. 6. Typical applications of the conductive Cu structures. (a) Flexible electrode[47]; (b) monitoring the finger movement using the strain sensor[44]; (c) response of the proximity/touch sensor to finger touch[71]; (d) planar antenna and its bandwidth[45]
4.2 功能性铜基结构的应用
激光直写制备的复合结构可以拓宽铜基结构的应用范围。当在闭合导电通路两端施加电压时,结构将在焦耳热效应的作用下升温。如
![激光直写功能性铜基结构的典型应用。(a)柔性加热器[47];(b)准固态电容器及其循环伏安曲线[46];(c)湿度传感器对呼吸频率的监测[48];(d)温度传感器及其温度响应曲线[84]](/richHtml/zgjg/2021/48/8/0802012/img_7.jpg)
图 7. 激光直写功能性铜基结构的典型应用。(a)柔性加热器[47];(b)准固态电容器及其循环伏安曲线[46];(c)湿度传感器对呼吸频率的监测[48];(d)温度传感器及其温度响应曲线[84]
Fig. 7. Typical applications of the laser written functional Cu-based structures. (a) Flexible heater[47]; (b) quasi-solid capacitor and its cyclic voltammogram[46]; (c) monitoring the breath rate using the strain sensor[48]; (d) temperature sensor and its temperature response curves[84]
5 结束语
总而言之,由于其无接触、无需掩模及加工快速的特点,激光直写已成为铜微纳结构的高效制造技术。基于离子态前驱体的激光直写整合了铜纳米材料的合成、定位、组装及连接,且在结构及成分的调控方面展现出独特的优势。目前,已掌握了在多种类型基板上可控直写铜基微纳结构的方法,且据此开发了多种微电子器件原型。应该指出的是,激光直写铜微纳结构仍然存在着亟待解决的问题:目前激光直写铜结构的研究主要仍针对纯铜结构展开,复合结构的制造方法尚不成熟;相较于分步制造方法,激光直写对铜材料的纳观结构(例如尺寸、均匀性)很难控制;虽可通过激光参数的调控对结构的成分(铜及铜氧化物)进行一定程度的调控,但所获铜结构通常为多组分复合结构。此外,一步法激光直写过程涉及到包含物理化学在内的多学科交叉,其直写机理尚未完全明确:如采用不同波长/类型激光直写所得结构的特征(成分、连接程度等)有待对比;直写过程中不同程度的热/等离子激元效应对所得铜结构的影响有待明确;直写过程中复合材料的还原、连接机理尚需深入研究;基于液态前驱体直写过程中流体的影响有待明晰。激光直写机理、直写结构的精细调控及复合铜基结构的制备将是未来相关研究开展的重要命题。再者,激光直写所得铜基结构的应用范围尚有扩展空间,直写结构的组织、成分及电性能对器件性能的影响尚待阐明,且器件的性能有待进一步优化。
[14] 高少华, 禹宣伊, 宋筱, 等. 基于飞秒激光直写的液晶面外区域定向技术及其应用[J]. 中国激光, 2019, 46(5): 0508009.
[15] 史杨, 许兵, 吴东, 等. 飞秒激光直写技术制备功能化微流控芯片研究进展[J]. 中国激光, 2019, 46(10): 1000001.
[19] 陈忠贇, 方淦, 曹良成, 等. 飞秒激光光镊直写银微纳结构[J]. 中国激光, 2018, 45(4): 0402006.
[22] Rajeeva B B, Wu Z L, Briggs A, et al. Direct-write printing: “point-and-shoot” synthesis of metallic ring arrays and surface-enhanced optical spectroscopy[J]. Advanced Optical Materials, 2018, 6(10): 1870038.
[24] Lü C J, Varanakkottu S N, Baier T, et al. Controlling the trajectories of nano/micro particles using light-actuated Marangoni flow[J]. Nano Letters, 2018, 18(11): 6924-6930.
[25] Lin L H, Peng X L, Mao Z M, et al. Bubble-pen lithography[J]. Nano Letters, 2016, 16(1): 701-708.
[43] Han S, Hong S, Yeo J, et al. Nanorecycling:monolithic integration of copper and copper oxide nanowire network electrode through selective reversible photothermochemical reduction[J]. Advanced Materials, 2015, 27(41): 6397-6403.
[44] Bai S, Zhang S G, Zhou W P, et al. Laser-assisted reduction of highly conductive circuits based on copper nitrate for flexible printed sensors[J]. Nano-Micro Letters, 2017, 9(4): 1-13.
[50] 廖嘉宁, 王欣达, 周兴汶, 等. 飞秒激光直写铜微电极研究[J]. 中国激光, 2019, 46(10): 1002013.
[57] Min H, Lee B, Jeong S, et al. Fabrication of 10 μm-scale conductive Cu patterns by selective laser sintering of Cu complex ink[J]. Optics & Laser Technology, 2017, 88: 128-133.
[58] Zehnder S, Lorenz P, Ehrhardt M, et al. Laser-induced processes on the back side of dielectric surfaces using a CuSO4-based absorber liquid[J]. Proceedings of SPIE, 2014, 8968: 896812.
[65] Kim T G, Park H J, Woo K, et al. Enhanced oxidation-resistant Cu@Ni core-shell nanoparticles for printed flexible electrodes[J]. ACS Applied Materials & Interfaces, 2018, 10(1): 1059-1066.
[66] Ye D M, Li G Z, Wang G G, et al. One-pot synthesis of copper nanowire decorated by reduced graphene oxide with excellent oxidation resistance and stability[J]. Applied Surface Science, 2019, 467/468: 158-167.
[73] Liu Y Q, Zhang Y L, Jiao Z Z, et al. Directly drawing high-performance capacitive sensors on copying tissues[J]. Nanoscale, 2018, 10(36): 17002-17006.
[75] Rahimi R, Es-haghi S S, Chittiboyina S, et al. Laser-enabled processing of stretchable electronics on a hydrolytically degradable hydrogel[J]. Advanced Healthcare Materials, 2018, 7(16): 1800231.
[84] Mizoshiri M, Ito Y, Arakane S, et al. Direct fabrication of Cu/Cu2O composite micro-temperature sensor using femtosecond laser reduction patterning[J]. Japanese Journal of Applied Physics, 2016, 55(6S1): 06GP05.
Article Outline
周兴汶, 廖嘉宁, 姚煜, 康慧, 郭伟, 彭鹏. 铜微纳结构的激光直写及其应用研究进展[J]. 中国激光, 2021, 48(8): 0802012. Xingwen Zhou, Jianing Liao, Yu Yao, Hui Kang, Wei Guo, Peng Peng. Direct Laser Writing of Micro/Nano Copper Structures and Their Applications[J]. Chinese Journal of Lasers, 2021, 48(8): 0802012.