MBE脱氧条件与InGaAs/InP APD性能的相关性【增强内容出版】
1 Introduction
The III-V semiconductor material InGaAs has a direct band gap and high optical absorption efficiency in the near-infrared band,which can be matched with the lattice of InP materials with good collisional ionization properties as one of the replaceable indirect band gap silicon-based materials[1]. Avalanche photodiodes(APDs)fabricated of InP and InGaAs materials for quantum key distribution[2],deep-space communications[3],3-D light detection and ranging[4,5],etc.,have many advantages such as high performance,high reliability,and low cost making a promising development outlook as optical signal detector applications. However,the performance of APDs is often limited by lattice defects in InP and InGaAs materials and the process conditions as the improvement of the structures. Therefore,preparing high-performance InGaAs/InP APD material remains challenging for researchers.
Molecular beam epitaxy(MBE)technology which has become more advanced in recent years,can control the parameters in the material deposition process,such as temperature,gas flow rate,sputtering,and deoxidation conditions,and precisely control the material thickness at the atomic layer scale,thus effectively regulating the material crystal structure and properties such as mobility,dark current and light saturation current[6,7]. In the MBE process,the atmosphere of evaporative sources is used to remove natural oxides from the substrate surface and protect against As evaporation damage,thus avoiding pollution from oxygen and other gases,known as deoxidation protection[8,9]. However,the effects of deoxidation atmospheres such as As and P sources on the performance of materials and devices,like impurities at arsenic-phosphorus heterojunctions,still need further research and optimization.[10,11].
In this paper,InP substrates were deoxidized under the atmosphere protection of As4 and P2,respectively. An ultra-high vacuum solid source molecular beam epitaxy(MBE)system grew lattice-matched In0.53Ga0.47As film and APD full-structure materials. The material minority carrier lifetimes were characterized by time-resolved photoluminescence(TRPL). The variation of As and P atoms and impurity concentration with thickness at the material structure interface were gauged by SIMS. The fluctuation of the In0.53Ga0.47As/InP heterojunction interface and necessary geometrical information is observed by scanning transmission electron microscopy(STEM). Finally,planar APD devices were prepared,and the IV characteristics were measured.
The effect of As-source deoxidation conditions is superior to that of P-source deoxidation conditions in terms of material surface morphology,lattice quality,minority carrier lifetime,and dark device current. This work offers a practical foundation for identifying optimal epitaxial growth and device processing parameters for InP-based InGaAs/InP.
1 Experiments
1.1 Material growth
The left side of
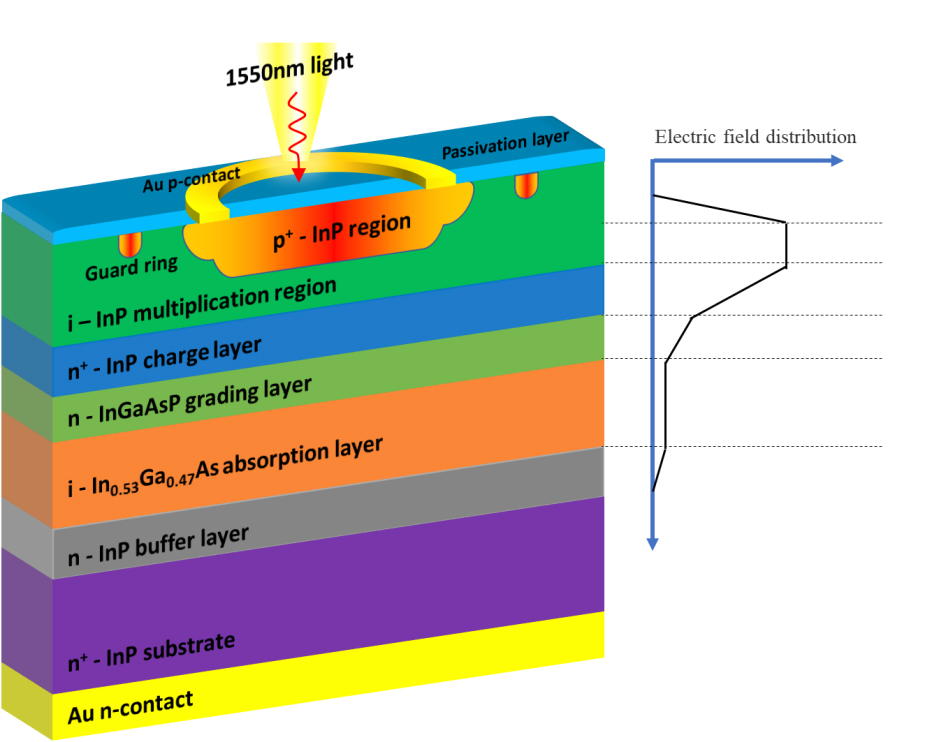
图 1. InGaAs/InP APD结构的剖面图和内部电场分布
Fig. 1. Profile diagram and internal electric field distribution of InGaAs/InP APD structure
The InP substrate was first put into the load lock and baked at 200°C to remove the adsorbed water vapor and then degassed in a pretreatment chamber at 300°C for 20 min. In the growth chamber,the intensity of the beam flux of each source at different temperatures was corrected to determine the growth temperature of each source. The beam flux ratios of group V and group III elements were determined using the beam flux monitors(BFM). The substrate was then transferred to the growth chamber. The substrate temperature of sample A was elevated under the protection of the As4 atmosphere,and sample B was performed under the P2 atmosphere to desorb the oxides from the surface. A clear 2×4 reconstitution stripe appeared on the reflection high-energy electron diffraction(RHEED)fluorescence screen,indicating desorption of the oxide layer on the substrate surface[9]. Afterward,the sample holder temperature continued to be raised by 30°C(thermocouple temperature ~640°C)and maintained for 6-7 min to allow complete desorption of the oxide film and flattening of the atomic layer on the substrate surface. After cooling down to the growth temperature,the beam equivalent pressure(BEP)ratios of each beam source furnace were controlled by valves to epitaxially grow 1~2 μm lattice-matched unintentionally doped In0.53Ga0.47As/InP film materials,and APD structured wafers. The APD material was based on a separate absorption,grading,charge,and multiplication(SAGCM)structure,including an n-InP buffer layer,an intrinsic In0.53Ga0.47As absorber layer,an InGaAsP component gradient layer,an n-type highly doped InP charge control layer,intrinsic InP cap layer,divides into Sample A(As4 deoxygenated)and Sample B(P2 deoxygenated)[4].
1.2 Material characterization
After growth,the materials were characterized using XRD to determine the component values and the lattice quality. Van der Pauw method Hall test(Ecopia HMS-3000)was performed to characterize the carrier transport properties of epitaxial In0.53Ga0.47As/InP materials of different thicknesses.
TRPL can characterize the direct electron-hole recombination(band-to-band recombination),Shockley-Read-Hall(SRH)recombination,and other non-radiative recombination information of materials absorbing pulsed laser radiation. [12]. The time-correlated single-photon counting(TCSPC)is a crucial technique for fluorescence lifetime imaging due to its high signal-to-noise ratio and high temporal resolution[13]. The dynamics of carrier recombination in APD structure materials were studied using the TCSPC method with a 1064 nm laser,and the results were compared with samples from the anti-reflective coating(ARC)SiNx passivation treatment(front-end process)[14]. The single-photon detector is a Hamamatsu H10330C -75 microchannel plate detector with a time resolution of 20 ps.
The SIMS principle is to use a focused ion beam sputtering the surface of semiconductors and then use a time-of-flight or mass spectrometer to analyze the secondary ions formed in the sputtering process,which allows for high-precision detection of the elemental distribution and interface impurities in the film materials from nanometer to tens of microns in depth[15,16]. We used the SIMS(Cameca IMF 5F,Evans Analytical Group)test to observe the high-precision measurement of different elemental concentrations in structural samples to analyze and determine the growth behavior and quality of InGaAs lattice epitaxy on InP substrates. The detection limit(DL) is over 1.0×1010 to 1×1016 atoms/cm-3.
Interfaces and defects in MBE-grown InGaAs/InP films were further examined using aberration-corrected STEM. Cross sections of STEM sample A(As4 deoxygenated)and sample B(P2 deoxygenated)were prepared using the focused ion beam(FIB)technique(Helios-G4-CX,Thermo Fisher Scientific). The STEM investigations were performed with a field-emission STEM(Themis Z,Thermo Fisher Scientific)fit with a probe and an image aberration corrector(SCORR and CETCOR,CEOS GmbH). The microscope was operated at 300 kV. For HAADF-STEM imaging,the probe semi-convergent angle was set to 21.4 mrad,and the semi-collection angle of the detector was arranged from 76 to 200 mrad. The STEM images were collected with[101]zone axis.
1.3 Device preparation and characterization
After characterization,the incident surfaces of APD materials were coated with SiNx ARC to eliminate reflections from the air-InP interface. The wafers were fabricated into 20 µm diameter planar InGaAs/InP APD by front-end processes such as Zn doping and UV lithography,including Device A(As4 deoxygenated)and Device B(P2 deoxygenated)[11,17]. The IV characteristics were measured by a semiconductor parameter analyzer(Keysight 4200)at room temperature.
2 Results and discussions
2.1 Hall effect analysis
2.2 TRPL analysis
The smoother the decay of the measured TRPL curve,the larger the τ value,indicating the stability of wafer quality. The smaller the variation of the τ value,the better the wafer quality. We can see that As4 deoxidation has a longer minority carrier lifetime t2 than P2 deoxidation,indicating that the wafer of As4 deoxidation is of better quality than that of P2 deoxidation,with relatively fewer lattice defects and impurities and less radiation recombination. The t1 of the samples is tiny,which indicates that the carriers in the wafer are rapidly quenched after capture,with strong non-radiative recombination,and there may be many deep energy level recombination with high doping concentrations or background concentrations.
2.3 SIMS analysis

图 4. (a)通过 As4、P2 脱氧样品的SIMS深度分布对比,(b)深度d = 4.8-5.6 μm的脱氧界面对比
Fig. 4. (a) Comparison of SIMS depth distributions for Samples via As4, P2 deoxidation,(b) Bulk regions (d = 4.8-5.6 μm) of comparison of deoxidation interface
表 1. SIMS测量的(d = 4.8-5.6μm)的区域杂质平均最大浓度(cm-3)
Table 1. Average peak concentrations (cm-3 ) of impurities in bulk regions (d = 4.8-5.6 μm) from SIMS measurements.
|
2.4 STEM analysis
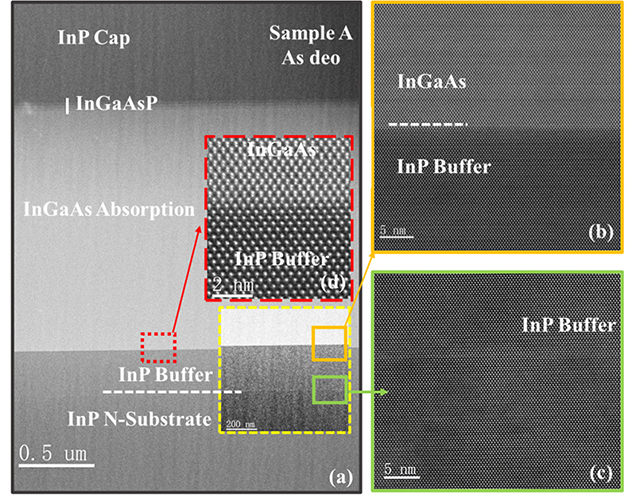
图 5. APD 样品A的STEM-HAADF图像(As-脱氧)(a)FIB网格上的APD结构图像,(b)InGaAs和InP缓冲层的图像,(c)InP同质结界面的区域,(d)(a)中红框区域的高分辨率图像
Fig. 5. STEM-HAADF image of APD sample A(As-deoxidation)(a)an image of the APD structure on FIB grid,(b)the image of InGaAs and InP buffer,(c)zoom region of InP homojunction interfaces,(d)high-resolution images of the region marked with a red frame in(a)
As shown in
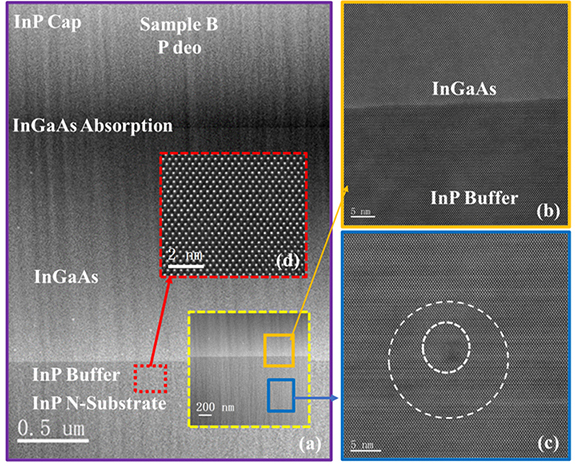
图 6. APD样品B的STEM-HAADF图像(P-脱氧)(a)FIB网格上的APD结构图像,(b)InGaAs和InP缓冲层的图像,(c)InP同质结界面的区域和堆叠缺陷(圆圈)圆圈表示具有不同核心结构的位错,(d)(a)中红框区域的高分辨率图像
Fig. 6. STEM-HAADF image of APD sample B(P-deoxidation)(a)an image of the APD structure on the FIB grid,(b)the image of InGaAs and InP buffer,(c)the zoom region of InP homojunction interfaces and stacking fault(circled). Circles indicate dislocations with different core structures,(d)high-resolution images of the area marked with a red frame in(a)
2.5 Device IV analysis

图 7. (a)InGaAs/InP APD的I-V曲线,(b)经钝化处理的APD材料TRPL光谱
Fig. 7. (a) I-V and gain characteristics of the InGaAs/InP APD,(b) TRPL spectra of ARC-APD
Compared to device A(As4 deoxidation),device B(P2 deoxidation)has a lower punch-through voltage,a slightly higher breakdown voltage,and a more extended linear region(less than 1 V). This is mainly due to the small thickness difference of the InP multiplication layer[28,30]. The elevated bias voltage increases the tunneling current in the absorption layer,which can lead to a significant increase in the dark current,thus affecting the performance and power consumption of the device. We note that near the punch-through voltage,the dark current of device B is 2.62×10-9 A,an order of magnitude larger than the dark current of device A of 1.62×10-10 A. From
The carrier lifetime measured for wafers is not the bulk carrier lifetime
Combined the IV analysis with the above Hall test and TRPL results,the APD with As4 deoxidation has lower carrier concentrations,higher Hall mobilities,and higher minority carrier lifetimes compared to the P2 deoxidation,which produces some suppression of the dark current caused by point defects or impurity defect energy levels. The SIMS and STEM results also support this view further.
3 Conclusions
In summary,we used the MBE technique to deoxidize InP substrates under As4 and P2 atmosphere protection and grow In0.53Ga0.47As/InP epitaxial layer APD structures. The material quality is evaluated by STEM,TRPL,Hall test,SIMS,and other characterization methods. The APD devices were prepared by a front-end process. The IV characteristics were measured at room temperature. The effects of MBE deoxidation conditions on the material surface morphology,lattice quality,minority carrier lifetime,and dark device current were analyzed. The results show that compared with P2 deoxidation,As4 deoxidation can significantly improve the MBE material quality,make the heterogeneous interface structure stable and sharp,improve theHall mobility,reduce thecarrier concentration,lengthen the minority carrier lifetime,and have a specific suppression effect on the device dark current caused by point defects or impurity defects. This study provides valuable information on InP/InGaAs APD material devices prepared by MBE.
[1] 李雪, 龚海梅, 邵秀梅, 等. 短波红外InGaAs焦平面研究进展[J]. 红外与毫米波学报, 2022, 41(1): 129-138.
[7] 杨瑛, 王红真, 范柳燕、, 等. 分子束外延高铟组分InGaAs薄膜研究[J]. 红外与毫米波学报, 2022, 41(6): 987-994.
YANG Ying, WANG Hong-Zhen, FAN Liu-Yan, et al. Study on Molecular Beam Epitaxy of High indium InGaAs Films[J]. J. Infrared Millim. Waves, 2022, 41(6): 987-994.
Article Outline
郭子路, 王文娟, 曲会丹, 范柳燕, 诸毅诚, 王亚杰, 郑长林, 王兴军, 陈平平, 陆卫. MBE脱氧条件与InGaAs/InP APD性能的相关性[J]. 红外与毫米波学报, 2024, 43(1): 63. Zi-Lu GUO, Wen-Juan WANG, Hui-Dan QU, Liu--Yan FAN, Yi-Cheng ZHU, Ya-Jie WANG, Chang-Lin ZHENG, Xing-Jun WANG, Ping-Ping CHEN, Wei LU. Correlation between MBE deoxidation conditions and InGaAs/InP APD performance[J]. Journal of Infrared and Millimeter Waves, 2024, 43(1): 63.