Efficient 671 nm red light generation in annealed proton-exchanged periodically poled LiNbO3 waveguides
Download: 690次
Coherent red light sources at around 671 nm have important applications such as full-color laser display[1,2], optical cooling and trapping of lithium atoms[3], and generation of entangled beams in quantum information technology[4]. Frequency doubling of neodymium-doped solid-state lasers is a conventional approach to obtain 671 nm laser light sources, and nonlinear crystals based on the quasi-phase-matching technique are commonly used due to the high-frequency conversion efficiency. Generation of red light at 671 nm based on second-harmonic generation (SHG) using quasi-phase-matched bulk crystals has been demonstrated, such as single-pass SHG using periodically poled stoichiometric [5,6] and extra-cavity frequency doubling with periodically poled [7]. Nonlinear interactions can be more efficient in waveguides compared with that in bulk crystals, because the light field is confined in a small cross section. In addition, high optical intensity is maintained over a long propagation length without divergence by diffraction, and thus efficient frequency conversions can be achieved in a single-pass configuration, reducing the complexity of the optical setup as compared to extra-cavity frequency conversions. There are several techniques to obtain the waveguide structure in , such as annealed proton exchange (APE)[8], Ti in-diffusion[9], and optical grade dicing[10,11]. APE waveguides show low propagation loss and fine nonlinear performance due to the annealing process. Besides, comparing with optical grade dicing, where the high-precision dicing technique is needed, the fabrication process of APE is relatively simple. High-performance quasi-phase-matched second-order nonlinear interactions have been demonstrated in APE periodically poled (PPLN) waveguides, such as generation of high-brightness entangled photons[12], enhanced electro-optic spectral tuning device[13], and efficient third-harmonic generation in the communication band[14].
In this work, we designed and fabricated APE PPLN waveguides for 671 nm red light generation and characterized the SHG performances of the nonlinear waveguides. The normalized SHG efficiency was for continuous-wave (CW) input at 1342 nm. In addition, when the quasi-continuous fundamental wave (FW) with a peak power of 2 W was used, the conversion efficiency was 60%.
To design the APE PPLN channel waveguides, the geometric structure and the poling period of the waveguide are the key parameters. Since the proton-exchange process only increases the extraordinary refractive index (), only TM modes are supported in z-cut APE waveguides. The extraordinary refractive index change of the APE waveguide can be described as , where is the wavelength-dependent coefficient, and is the normalized proton concentration[15]. The profile of is determined by the channel width W and the annealing depth D[16], and the annealing depths in the direction and direction were assumed to be the same for simplicity. To obtain low propagation loss, the surface proton concentration should be smaller than 0.23[16], and thus a relatively large annealing depth is required to support the guide mode in the near-infrared spectral range. The single-mode condition for the FW at 1342 nm was estimated as follows: , , and . The simulated refractive index increment of the mode at 1342 nm and 671 nm is shown in Fig.

Fig. 1. (a) Refractive index increment as a function of surface proton concentration in 6 μm wide channel APE waveguides with annealing depth of 3 μm. (b) Theoretical poling period for our APE PPLN structure as a function of temperature, where the surface proton concentration varies from 0.12 to 0.16.
The detailed fabrication process for the channel APE PPLN waveguide is described as follows. Full-wafer periodical poling was obtained using the conventional electric field technique at room temperature[18]. Then a 200-nm-thick film was deposited on the PPLN wafer using plasma-enhanced chemical vapor deposition. Subsequent UV lithography and inductively coupled plasma etching were used to form the mask with open channels, and the channel waveguide structure was defined by the mask. For the APE channel waveguide, the waveguide pattern is defined by the mask, and thus the line edge roughness of the mask will transfer to the waveguide. To reduce the waveguide propagation loss caused by interface scattering[19], a fine-quality mask is required. From the scanning electron microscope (SEM) picture of the mask shown in Fig.

Fig. 2. (a) SEM picture of mask. (b) Image of the inverted domain structure of the APE waveguide recorded using confocal SH microscopy. (c) The measured and fitted transmission spectra of the waveguide.
The schematic experimental setup for SHG of red light at 671 nm is shown in Fig.
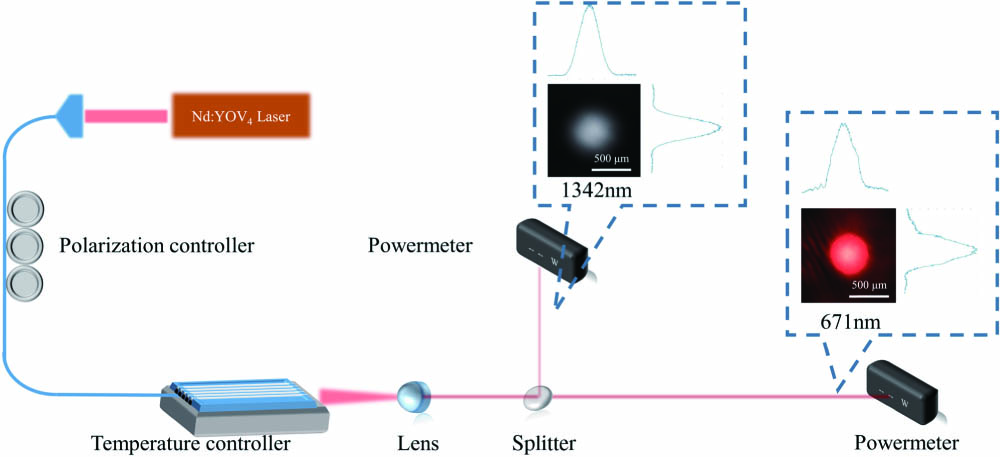
Fig. 3. Schematic experimental setup. The two insets are the images of the FW and SH wave, respectively.
Firstly, we turned off the Q-switch, the fundamental laser light source was in a CW operation mode, and we tested the single-pass SHG performance of the nonlinear waveguides at 1342 nm. SHG was achieved in the waveguides with poling periods of 11.78 and 12.02 μm, and the corresponding quasi-phase-matching temperature was measured to be 141.0°C and 40°C, respectively. According to the measured quasi-phase-matching temperature, the surface proton concentration was estimated to be 0.12, which was used to calculate the theoretical frequency conversion efficiency of the fabricated sample. We chose the waveguide with higher quasi-phase-matching temperature, and the temperature tuning curve of this waveguide is shown in Fig.

Fig. 4. Temperature tuning curve of APE PPLN waveguide with 11.78 μm poling period. The black dots and the dashed red line are the measured data and fitted curve, respectively.
The SHG conversion efficiency as a function of the input power of the FW is shown in Fig.

Fig. 5. Measured SHG conversion efficiency as a function of the power of FW with (a) CW and (b) 50 ns, 10 kHz pulsed input. The blue dots and the green line correspond to the measured conversion efficiency and the linear fitting curve, respectively.
To conclude, we have designed and fabricated periodically poled channel APE waveguides for efficient 671 nm red light generation. The channel waveguides were fabricated on PPLN by the APE technique. By optimizing the fabrication process, the PPLN channel waveguides with 0.097 dB/cm low propagation loss were obtained, and the waveguide exhibited excellent SHG performance. With CW input, 1.71 mW red light was obtained with normalized efficiency up to , which is nearly 90% that of the theoretical value. The SHG conversion efficiency reached up to 60% when pulsed FW with an average power of 1 mW was coupled into the waveguide. Our work provides a scheme for high-efficiency red light generation. To further raise the power-handle capacity of the APE periodically poled waveguide, MgO-doped can be used as the host material[27,28], which possesses higher photorefractive damage threshold.
[1]
[3]
[4]
[5]
[6]
[9]
[10]
[11]
[12]
[13]
[14]
[16]
[17]
[18]
[19]
[20]
[21]
[22]
[23]
[24]
[25]
[26]
[27]
[28]
Yunfei Niu, Lei Yang, Dongjie Guo, Yan Chen, Xiaoyang Li, Gang Zhao, Xiaopeng Hu. Efficient 671 nm red light generation in annealed proton-exchanged periodically poled LiNbO3 waveguides[J]. Chinese Optics Letters, 2020, 18(11): 111902.