Dielectric metasurface evolution from bulk to monolayer by strong coupling of quasi-BICs for second harmonic boosting
1. INTRODUCTION
As a widely observed nonlinear effect of optics, second harmonic generation (SHG) has been extensively applied in spectroscopy, sensing, and image processing [1–4]. Conventionally, there are several bulk materials used to build the optical devices of SHG, such as lithium niobate () and gallium arsenide (GaAs) [5–7]. In the past few years, 2D materials are found as promising multilayer or monolayer materials for constructing on-chip SHG devices, since they have the considerable nonlinear susceptibility in an ultrathin thickness (from several angstroms to several nanometers) [8–10]. Typically, such a small thickness makes the weak interaction between 2D materials with the incident light, and thus it requires the light trapping structure to enhance the localized optical near field significantly [11]. In view of this motivation, researchers have investigated the use of various plasmonic or dielectric metasurfaces for elevating the SHG responses of 2D materials [12–15]. Compared with the plasmonic counterparts, dielectric metasurfaces are much more attractive for efficient enhancement of nonlinear interaction in 2D materials, because they have much lower intrinsic optical losses governed by radiation leakage only [16–18] and provide higher quality factor (-factor) responses [18–20]. Particularly, by introducing the symmetry breaking, dielectric metasurfaces can exhibit the quasi-bound states in the continuum (q-BICs) [21,22] and have the potential to accomplish an extremely high -factor for the dramatical enhancement on SHG of 2D materials. So far, employing q-BICs of dielectric metasurfaces has been becoming the research hotspot on nonlinear optics of 2D materials [23,24]. However, most existing studies focus on designing the q-BIC dielectric metasurfaces with asymmetric nanostructure units of bulk materials, and combine them with 2D materials to enhance the SHG effect [23,25]. Such bulk metastructure design has a thickness with a giant difference from that of 2D materials. Its larger thickness exhibits distinct out-of-plane asymmetry, which induces considerable optical dissipation of radiation [26,27]. This not only suppresses the high -factor resonance performance of the metasurface, but also hinders efficient light field concentration on 2D materials. Very recently, researchers have found that one can dramatically lower the optical dissipation by reducing the patterned layer thickness of a dielectric metasurface from the bulk to the sheet [28,29], which might be promising for enhancing the SHG of 2D materials. Despite this, there is a critical limitation on ultrathin nanopattern fabrication of bulk metastructures [30]. Therefore, it is still essential to pursue a feasible mechanism based on the q-BIC dielectric metasurface for boosting the SHG of 2D materials.
Herein, we propose the design of a dielectric q-BIC metasurface based on the evolution from bulk material to monolayer material. We adopt the meta-atom unit of monolayer molybdenum disulfide () under a specific geometrical asymmetry, which supports two q-BIC modes with much higher -factors than the traditional bulk silicon (Si) metasurface design. By tuning the incident angle of the incident light, we obtain a resonance response with the extremely high -factor. It is attributed to the strong coupling of two q-BIC modes. The intensive near-field enhancement supported by such a strong coupling mechanism boosts the SHG of monolayer . Our study will provide a theoretical guide for the development of high-performance nonlinear photonic devices based on 2D materials.
2. DESIGN AND METHODS
The evolution from bulk to monolayer metasurfaces is shown in Fig. 1. There are three geometric manipulation mechanisms, namely, symmetrical breaking, thickness transformation, and angle control. The in-plane asymmetry configuration of a metasurface enables two q-BIC modes. Based on such asymmetry, we implement the transformation from bulk Si to sheet Si and monolayer . In our design, the monolayer is adopted as a nonlinear optical material, which has a large nonlinear susceptibility tensor of in the visible and near-infrared regions [31]. The meta-atoms of these metasurfaces are asymmetric rectangular pairs of bulk Si, sheet Si, and monolayer , respectively. They also consist of a silicon nitride () waveguide layer with a thickness of and the silicon dioxide () substrate. For the asymmetric pair of monolayer metasurfaces, we provide a feasible nanofabrication process (see details in Appendix A). The periods of metasurfaces along the - and -directions are and , respectively, where . The rectangular lengths in the -direction for each asymmetry pair are (, where represents the -direction rectangular width) and , respectively, while the asymmetry parameter is defined as . The thicknesses of the bulk Si, sheet Si, and are denoted as , , and , respectively. According to the literature, the refractive indexes of and are assumed as 2.02 and 1.45, respectively [32]. The complex refractive index parameters of Si, bulk, and monolayer are based on the experimental data [33,34] (see details in Appendix B). Particularly, the monolayer has a high refractive index with the infinitesimal extinction coefficient in this spectral range [35].
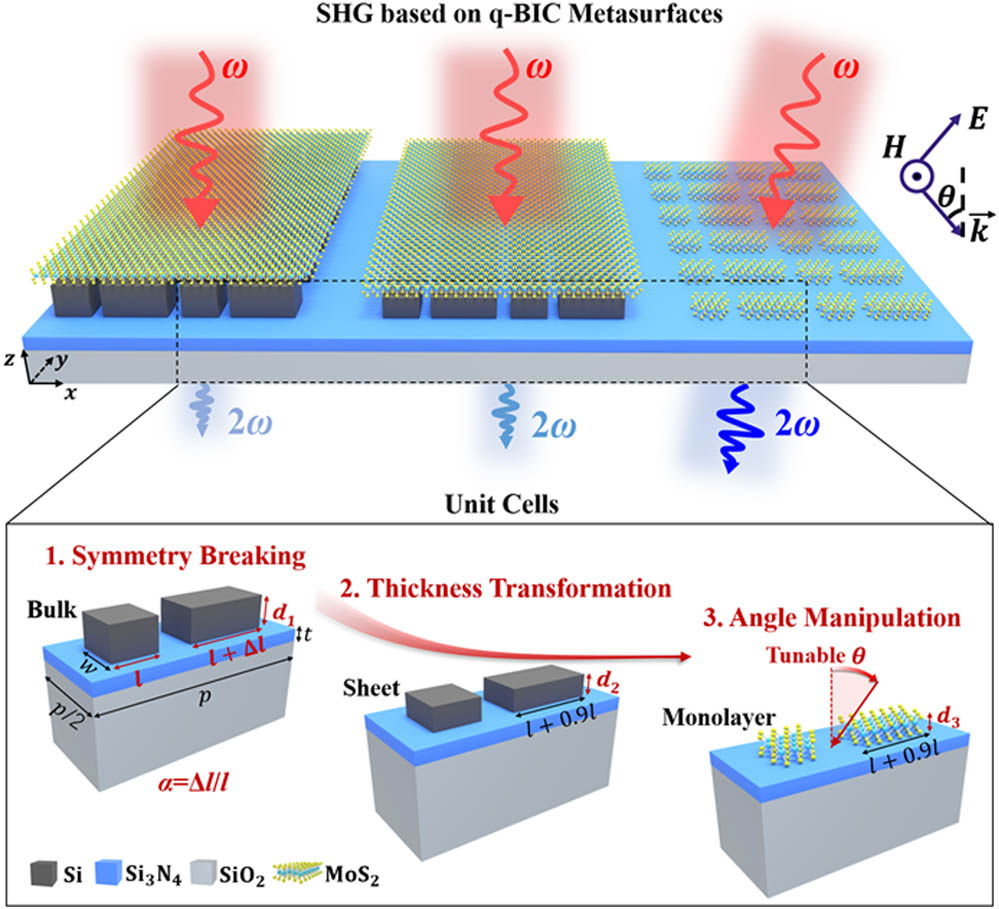
Fig. 1. Schematic diagram for the evolution from bulk to monolayer q-BIC metasurfaces, where the symbols , , , , , , and denote the period, thickness of waveguide layer, width and heights of various asymmetrical rectangular pairs, and the incident angle, respectively.
With the aim of studying the optical responses of metasurfaces, we employ the simulation modules for nonlinear optics by the commercial software COMSOL Multiphysics, which is based on the finite element method. In the simulation, Floquet boundary conditions are adopted on the unit cells of metasurfaces in - and -directions. The wave ports are used at the top and bottom of -direction. The optical incidence is applied from the top side of each meta-atom with the incident angle of and the intensity of . A full-wave simulation is conducted for the linear optical process, in which the localized near-field distributions of the fundamental wavelength are recorded and used to determine the nonlinear polarization as below [36]:
3. RESULTS AND DISCUSSION
3.1 A. High-Q Metasurfaces Empowered by In-Plane Asymmetry q-BIC and Thickness Evolution
We first investigate the optical properties of bulk Si metasurfaces with in-plane symmetry breaking. Their transmittance spectra demonstrate two resonances marked as and , respectively, as observed in Fig. 2(a). When changes from 1 to 0.05, the two resonances have the blue shift with a reduced full width at half maximum (FWHM), which indicates the variation tendency towards an extremely high -factor (, where denotes the resonance wavelength). As equals zero, the FWHMs of two resonances disappear, indicating two symmetry-protected BICs [38]. Here, the -factors of the two q-BIC modes can be fitted with (see details in Appendix C). Such property is also observed in some previous studies [18,27]. In the resonance system of an all-dielectric metasurface, a high- response implies the good capability to support high optical field enhancement [25], which is quite beneficial to strengthen the effects of nonlinear materials. In addition, the -factor is determined by the total rate of optical loss () in a q-BIC system, where , is the radiative loss, and represents the parasitic loss. Ideally, the dielectric metasurfaces are assumed to have negligible parasitic loss () [39], while the radiative loss increases quadratically with the asymmetry parameter, namely, [23]. Therefore, one can dramatically raise the -factor by decreasing the asymmetry factor at the state of q-BIC [39,40].

Fig. 2. (a) Transmittance of Si metasurface with in-plane asymmetric q-BIC as a function of , where . (b) Transmittance of Si metasurface with in-plane asymmetric q-BIC as a function of , where . (c) Transmittance of metasurface as a function of thickness , where . (d) Electric field distributions of metasurfaces for different thicknesses at the resonance wavelengths of . For all the figures, .
Since achieving the high- response is crucial for enhancing nonlinear effects, we continue to pursue another way to elevate the -factor of metasurfaces. As shown in Fig. 2(b), we fix the in-plane asymmetry of for the two above-mentioned q-BIC modes, and study the influence of asymmetry pair thickness on the -factor. It is observed that the -factors of both q-BIC resonances for are much larger than those for . In fact, the -factors of the two q-BIC modes can be described as the two functions of , respectively (see details in Appendix C). They demonstrate the relationship of between the -factor and asymmetry pair thickness. This is similar to the correlation between the -factor and asymmetry factor. It is because the reduction of asymmetry pair thickness lowers the radiative loss. The asymmetry pair thickness can be used as a tuning factor like to manipulate the radiative loss of q-BIC[29], that is, . For the thick asymmetry pair with large , the -factor of q-BIC is limited by higher radiative losses due to distinct out-of-plane asymmetry. By thinning from 100 nm to 10 nm, the volumes of each asymmetry pair progressively decrease. Such reduction will suppress the radiation channel propagating outside the system, leading to a substantial decrease in radiative loss. As a result, when (, the -factors can reach up to 13,751 and 14,254, which are higher than those obtained when () (see details in Appendix D). This result proves that the asymmetry pair thickness evolution can be also used as a vital factor to increase the -factor of q-BIC in addition to tuning the in-plane asymmetry factor.
Although the use of traditional Si is readily available, the fabrication of its 2D counterpart still faces great challenges [30]. As a natural 2D material, can be easily accessed to the monolayer thickness. Therefore, we next focus on using as the material of asymmetry pairs instead of Si. The simulated transmittance spectra for various thicknesses in Fig. 2(c) also show two resonance modes. When is changed from 50 nm to 0.65 nm at the fixed value of 0.9, the two q-BIC modes also show the trend of blue shift with dramatically increased -factors. This is due to the reduction of effective refractive index induced by thinning asymmetry pairs. For the metasurface of the monolayer (0.65 nm), the -factors of and can be up to and , respectively (see details in Appendix D).
The q-BICs with ultrahigh -factors are usually associated with the significant enhancement of the localized electric field surrounding the metasurface [41]. Such field enhancement should be beneficial to strengthen the nonlinear polarization, according to Eq. (1). In view of this, we plot the electric field distributions in Fig. 2(d) for metasurfaces with different thicknesses at the resonance wavelengths of and , respectively. The electric field distributions at indicate a typical feature of guided mode resonance (GMR), which are induced by the leaky guided mode [42]. At the resonance wavelength of , the presence of multiple interference modes in the waveguide layer indicates that it is the higher-order GMR mode [43]. Notably, decreasing the thickness of boosts the field confinement around the asymmetry pair of , as shown in Fig. 2(d). The electric field intensity for the monolayer is two orders greater than that of the 50 nm thickness. This significant enhancement of the localized near field indicates the much stronger light-matter interaction per unit area in the monolayer , which is critical to elevate the efficiency of energy utilization by nonlinear effects of .
We continue to investigate the influence of on the BIC-based -factors of monolayer metasurfaces in Figs. 3(a) and 3(b). Like the conventional Si counterpart, they have an increase of -factors with the decrease of for both and modes. The resonance dips of both q-BIC modes vanish when equals zero, suggesting the states of non-radiative BIC. We further calculate the -factors of the two resonance modes in Figs. 3(c) and 3(d), which can be also fitted with and demonstrate the physical features of q-BIC modes. Specifically, the -factors of the two q-BIC modes can both reach an ultrahigh level of for . Therefore, the tuning of the asymmetry factor could further elevate the -factors of q-BIC resonances based on monolayer metasurfaces, which would be promising to enhance the localized electric field like the thickness engineering of metasurfaces exhibited in Fig. 2(d).
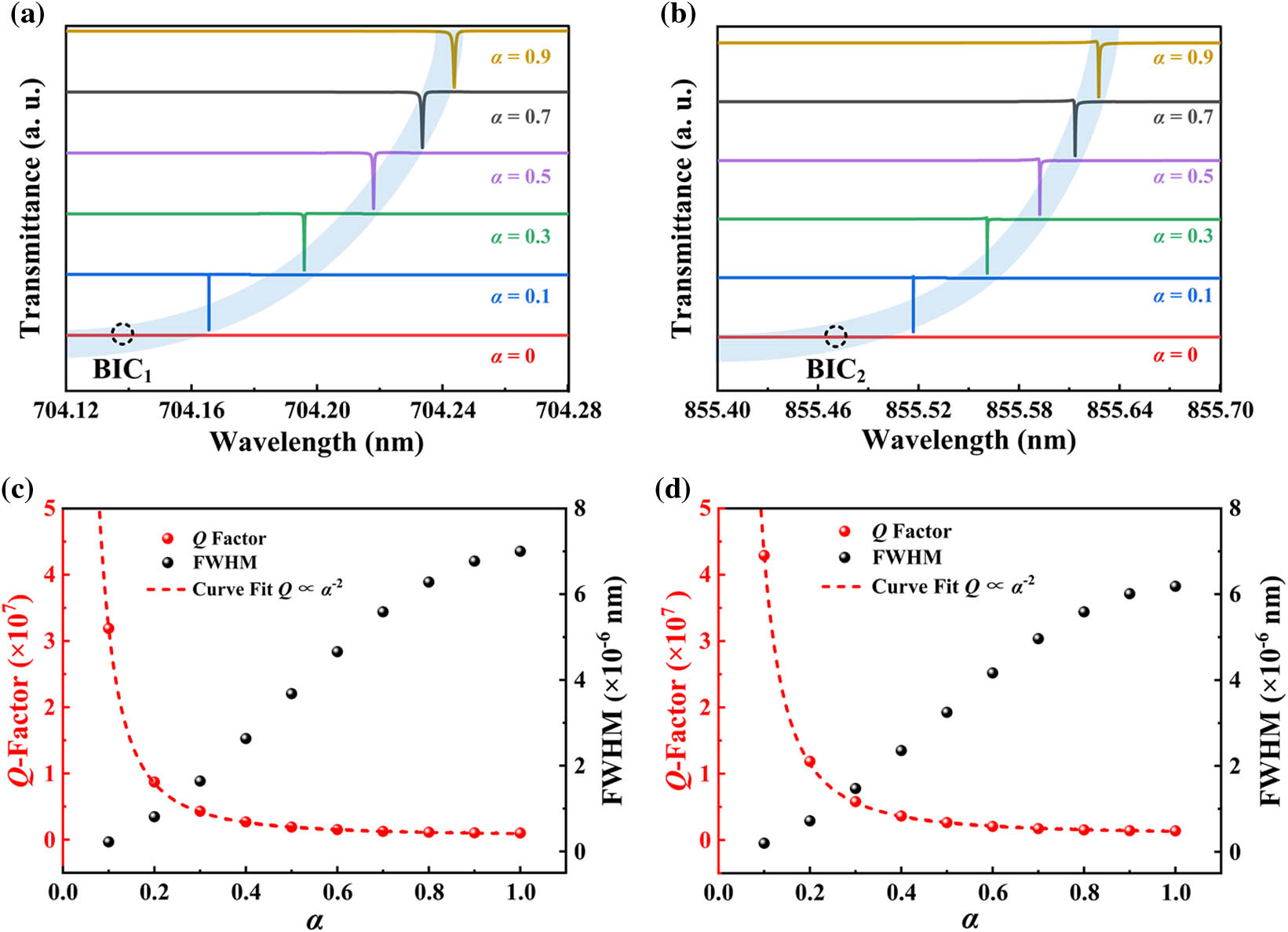
Fig. 3. (a), (b) Transmittance spectra of monolayer metasurface as changes from 0 to 0.9 for (a) and (b) . (c), (d) -factor and FWHM as functions of for (c) and (d) .
3.2 B. Metasurface of Monolayer Empowered by Strong Coupling of q-BICs
In the above discussion, the two high- resonances of q-BIC modes are achieved through the thickness evolution from bulk to monolayer metasurfaces and the tuning of the asymmetric factor. These two high- modes stem from the high-order and low-order GMR modes, respectively. Previous research has illuminated that neighboring GMR modes could have electromagnetic coupling under oblique incidence, which leads to a higher -factor of resonance [42]. Thus, we next investigate the coupling of the two q-BIC modes under oblique incidence in order to further boost the -factor for a higher localized electric field. As shown in Fig. 4(a), when the incident angle changes from 4° to 8°, the resonance wavelengths of and move towards the directions of long and short wavelengths, respectively, and get close to each other. In particular, when the incident angle increases to 10.5°, there is only one resonance feature observed on the spectrum around the wavelength of 776.505 nm. This is because the radiation feature of mode disappears and is transformed into the distinct feature of Friedrich-Wintgen BIC mode [44]. As the incident angle goes over 10.5° and increases from 13° to 17°, the two resonance features of and modes can be re-observed, and they continue to move towards the directions of long and short wavelengths. The angle-resolved transmittance spectra show an anti-crossing behavior denoted by the red and blue bands, which implies the strong coupling of the two q-BIC modes and can be described by the following equation [45]:

Fig. 4. (a) Resonance behavior of and as incident angle changes from 4° to 17°. (b) -factors of and with the change of . (c) Transmittance spectra and the corresponding electric field distributions for four incident angles of 6°, 10.4°, 10.5°, and 15°, respectively.
Based on Eq. (4), we can have the energy of Rabi splitting as below:
When the strong coupling of two q-BIC modes occurs, the following criteria should be satisfied [46,47]:
In this case, we have , , , and , which proves that it is a state of strong coupling.
We conduct a further evaluation of the -factor evolution of two q-BIC modes under oblique incidence, as illustrated in Fig. 4(b). As the incident angle increases from 0° to 10.5°, the -factor of exhibits a sharp increase towards infinity, while that of decreases gradually, reaching its minimum at 10.5°. To reveal the physics, we present an enlarged view of the transmittance spectra for various incident angles and the corresponding localized electric field distributions in Fig. 4(c). At the incident angle of 6°, the electric field distribution clearly exhibits a high-order mode feature at , whereas the low-order mode is observed at . When the angle is 10.4°, the two modes are very close to the state of Friedrich-Wintgen BIC and a clear mode hybridization is observed at the wavelength of 775.724 nm. This denotes the feature of strong coupling, leading to energy redistribution through the two modes. Consequently, the mode demonstrates the ultranarrow FWHM and ultrahigh electric field intensity, which are promising for the high-performance SHG device. At the incident angle of 10.5°, the destructive interference launches the dark mode, which collapses the radiation channel and prohibits the coupling to external radiation [48,49]. As the angle increases to 15°, the vanished near-field feature re-emerges, and the two modes undergo band flip, wherein their respective electric field distributions interchange. In combination with the tuning of on the strong coupling condition, we can further enhance the -factor to an extremely high level of (see details in Appendix D).
3.3 C. SHG Enhancement in Monolayer Metasurface
Based on the proposed monolayer metasurface with the ultrahigh -factor, we next evaluate its performance for nonlinear optics. We compare the SHG conversion efficiency of the monolayer metasurface to that of the Si metasurface coated by an unpatterned monolayer , as shown in Fig. 5. When the metasurface is excited by a pump laser with normal incidence, the SHG conversion efficiencies of monolayer metasurfaces for both and are about times higher than that of the Si metasurface. Particularly, when the incident angle is 10.4°, the two q-BIC modes are strongly coupled and the SHG conversion efficiency is dramatically enhanced, approaching 5.8% at the wavelength of 383.294 nm.
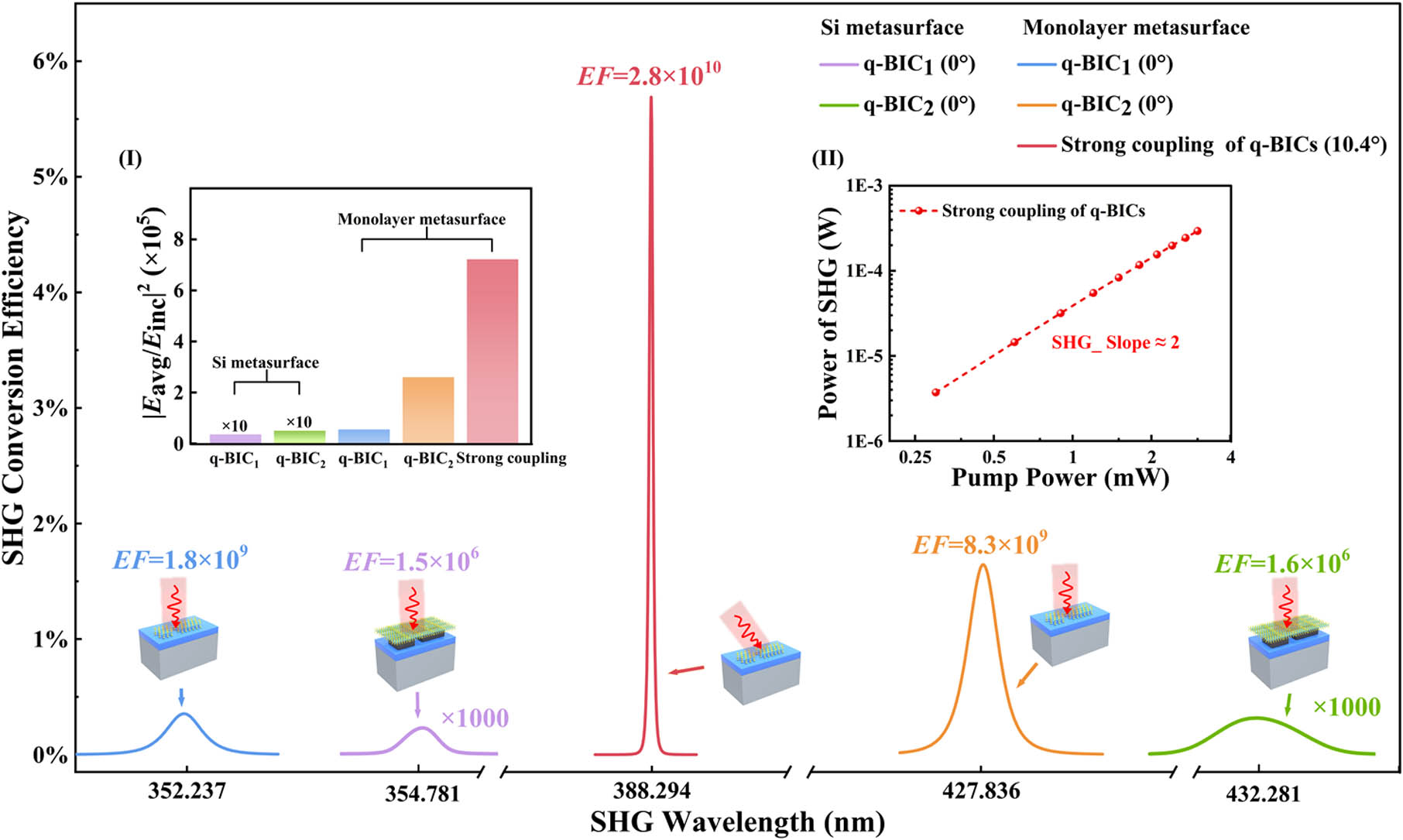
Fig. 5. SHG response and enhancement factor for monolayer metasurface and sheet Si (10 nm thick) metasurface with monolayer . Inset (I) denotes the average electric field enhancement factors for various modes. Inset (II) represents the power dependence of SHG in logarithmic scale. Here is fixed at 0.9.
In order to reveal the nonlinear optical effects, we use the enhancement factor (EF) to quantify the increase of SHG intensity on the metasurface in comparison with the natural homogeneous monolayer. The monolayer metasurface with strong coupling q-BIC exhibits a significantly increased EF of , which is times larger than that of the Si metasurface. Such high nonlinear performance is attributed to the electric field enhancement near the monolayer , as indicated in inset (I) of Fig. 5, which can be determined as below [50]:
Finally, we compare the SHG conversion efficiency and EF of the proposed monolayer metasurface with some pioneer studies, as presented in Table 1. Despite lower pump intensities, the EF of our metasurface is times larger than that of previous research using a Si metasurface, which implies its good nonlinear performance for future SHG. Existing SHG Conversion Efficiency on the Different StructuresNo. SHG Enhancement SHG Conversion Structures Refs. 1 0.5 27 — [51] 2 2.05 25 — [52] 3 2.4 68 — [37] 4 — Si with monolayer [53] 5 1 — Bulk [33] 6 7.2 80 565 — Two multilayer GaSe on top of [54]
4. CONCLUSIONS
In summary, we propose a dielectric metasurface empowered by two high- q-BIC modes, which are based on symmetry breaking and thickness transformation on the meta-atom. Particularly, along with the evolution from bulk Si to monolayer , the radiation loss of the metasurface is greatly reduced. We further utilize the strong coupling of the two q-BIC resonances by angle manipulation, and achieve an extremely high -factor up to , which also excites the exceptional near-field enhancement on monolayer . Our theoretical research elevates the SHG conversion efficiency significantly and will inspire more nonlinear optical studies of 2D materials using q-BICs.
5 Acknowledgment
Acknowledgment. We thank the National Model Microelectronics College at Xiamen University for providing equipment. In addition, Z.D. would like to acknowledge the funding support by Agency for Science, Technology and Research.
Article Outline
Yinong Xie, Qianting Chen, Jin Yao, Xueying Liu, Zhaogang Dong, Jinfeng Zhu. Dielectric metasurface evolution from bulk to monolayer by strong coupling of quasi-BICs for second harmonic boosting[J]. Photonics Research, 2024, 12(4): 784.