百瓦级中红外光纤随机激光放大
High-power laser sources operating in 2-μm band are gaining traction due to their diverse applications, including laser scalpels, plastic welding, and free-space laser communication. Over the past decade, random fiber lasers (RFLs) have emerged as a focal point of interest. These lasers utilize randomly distributed Rayleigh scattering and nonlinear amplification, distinguishing them from traditional resonant cavity lasers. The defining features of RFLs are their open cavity structure and incoherent feedback, which result in modeless lasing and significant suppression of temporal dynamics. However, research on RFLs has primarily concentrated on the near-infrared band, specifically around 1.1 μm and 1.5 μm, due to challenges such as significant transmission loss and weaker Rayleigh scattering in mid-infrared silica-based optical fibers. Historically, RFLs in the mid-infrared band have only realized low power outputs. In this study, we introduce a high-power RFL operating at 2 μm, utilizing the master oscillator power amplifier (MOPA) approach. Impressively, we realize a peak power of 100.40 W with an efficiency slope of 47.8% and a narrow 3 dB spectral width of approximately 0.2 nm.
Figure 1 depicts the experimental arrangement for a high-powered RFL using the MOPA design. The seed consists of a 793-nm laser diode (LD), a (2+1)×1 pump combiner, a 2.4-m long thulium-doped fiber, a 1980-nm FBG, a 200-m single-mode fiber (SMF), and an isolator (ISO). The 1980-nm FBG serves as a point reflector, selecting the wavelength and providing optical feedback, in tandem with the Rayleigh scattering in the extended SMF. An isolator at the SMF end prevents unwanted light reflection. The preamplifier stage consists of a 793-nm LD, (2+1)×1 pump combiner, a 2.4-m thulium-doped fiber, and an isolator. Its role is to enhance the seed laser output power to a specified threshold. Inserting a mode-field adaptor (MFA) between the preamplifier and main amplifier minimizes insertion loss due to mode mismatch among different fibers. The main amplifier takes the laser signal from the MFA and amplifies it using a (6+1)×1 combiner and 4.7-m long large-mode-area thulium-doped fiber (LMA-TDF). This configuration facilitates a lasing output in the 100-W range, powered by two 793-nm high-intensity LDs. To remove residual pump light, a cladding power stripper (CPS) is integrated at the LMA-TDF terminal. Its endpoint is oriented to counter unintended feedback from the Fresnel reflection. Lastly, all gain fibers are positioned on a water-cooled plate for thermal efficiency. In the main amplifier stage, these fibers are coiled to approximately 10 cm in diameter, mitigating high-order transverse modes.
The RFL seeds exhibit superior lasing characteristics, which encompass a narrow spectral linewidth and exceptional temporal stability. The threshold pump power for the seed source is determined as approximately 3.57 W, with a slope efficiency of 13.6%. The standard deviation divided by the mean value (A) stands at 0.0265 with a maximum pump power of 7.40 W. This is primarily attributed to the half-opened, non-resonant structure of the seed, in contrast to a resonant cavity (Fig.2). The preamplifier stage further boosts the output power. At a pump power of 8.96 W, the maximum output power from the preamplifier stage is 3.09 W, having a slope efficiency of 35.6%. Importantly, the output spectrum of the preamplifier stage retains its narrow linewidth feature. This is a benefit of the spectral-broading-free characteristic when the random fiber laser serves as the seed in the MOPA configuration (Fig.3). In the main amplification stage, a hundred-watt-level mid-infrared lasing output materializes. With a pump power of 215.7 W, the maximum output power corresponds to 100.40 W and demonstrates a slope efficiency of 47.8%. No decline in the output power is evident, and potential for further power enhancement is predominantly constrained by laboratory cooling conditions and available pump sources (Fig.4). Notably, the output spectrum of the main amplification stage also upholds a narrow linewidth, which benefits from the unique spectral broadening-free attribute of the power amplification process founded on a half-open-cavity seed. The RFL seed output power showcases extremely minimal power fluctuations, leading to suppressed nonlinear effects. Moreover, the inclusion of a large-mode-field gain fiber in the main amplification stage aids in curtailing nonlinear effects. There is no evident spectral broadening as the output power increases from 10.33 W to 100.40 W in terms of 3 dB and 10 dB bandwidths. The 3 dB spectral bandwidth at an output power of 100.40 W is approximately 0.2 nm (Fig.5). The final laser output also demonstrates remarkable stability in short and long durations. This stability arises from the suppressed temporal dynamics induced by the incoherent feedback process via randomly distributed Rayleigh scattering. The A value decreases from 0.1419 to 0.0319 as the output power increases from 20.00 W to 100.40 W (Fig.6). Furthermore, the output power fluctuation at approximately 72 W, in terms of the A value over a 30-min interval, is only 0.0048 (Fig.7).
In this study, a high-power RFL in 2-μm band is realized using the MOPA configuration. Due to the distinctive properties of the random laser, a lasing output with a remarkably narrow linewidth (approximately 0.2 nm) is obtained. The short-term domain dynamics and long-term power fluctuations of the lasing display excellent stability. This study offers a compelling alternative for crafting a high-performance random laser source in 2-μm band.
1 引言
近年来,光纤随机激光器(RFL)发展迅速[1-3]。区别于传统谐振腔型的光纤激光器,RFL中的开放腔结构和非相干反馈使得输出激光的时域动态无谐振纵模且强烈受抑制。目前,RFL的相关研究主要聚焦在高功率/高效率产生[4-7]、超宽带多波长激射[8-9]、窄线宽输出[10-11]、超连续谱产生[12-14]、分布式光放大[15]以及成像光源[16-17]等功能实现方面,已取得了一系列成果。值得注意的是,受限于中红外波段激光在石英光纤中的较大传输损耗和较弱的瑞利散射效应,RFL的工作波长仍主要集中在近红外波段(1.1~1.7 μm)[18-20]。因此,进一步拓宽光纤随机激光器的工作波长范围并提升其输出性能具有重要的科学意义和应用价值。
2 μm波段激光处于水分子吸收峰处,包含许多重要分子的特征谱线,在精密加工、环境检测、生物医疗等方面有着重要应用[21-24]。近年来,基于传统有源谐振腔的2 μm光纤激光器在高功率激光输出[25-26]、波长可调谐输出[27-28]、单频激光输出[29-30]等方面都取得了极大进展。但是,该腔型光纤激光器在结构紧凑性和输出激光时域稳定性等方面仍需进一步优化提升。近年来,深圳大学的Ma等[31]开展了基于无源反馈结构的中红外波段光纤随机激光器研究,对比了三种不同腔型结构下的2 μm光纤激光器,包括无源反馈半开放腔结构、单通放大结构和常规谐振腔结构,获得了时域动态极其稳定的随机激光输出。此外,中红外光纤随机激光器的相关研究还包括脉冲泵浦产生2.1 μm激光[32]、基于非线性频率转换产生中红外激光[9,33-34]、基于氟化物光纤的中红外随机激光的理论仿真和优化设计[35]以及基于光纤随机光栅产生中红外激光[36-37]等。已报道的相关研究工作所涉及的中红外光纤随机激光器的输出激光较弱。因此,须进一步提升中红外光纤随机激光器的输出功率以满足相关应用要求。本文提出了一种基于主振荡器功率放大器(MOPA)结构的2 μm高功率光纤随机激光器。激光器的最高输出功率为100.40 W,斜率效率为47.8%。同时,输出激光的短时时域和长时功率波动均表现出优良的稳定性。受益于MOPA结构中光纤随机激光种子源在激光放大过程中的光谱带宽保持特性,激光器在高功率运转时仍保持窄线宽输出特性。研究结果为2 μm高功率光纤随机激光器的优化设计提供了思路,并有望进一步拓展其应用范围。
2 实验结构
基于MOPA结构的1980 nm光纤随机激光器的实验装置如
3 实验结果与讨论
首先,开展了光纤随机激光器种子源特性研究。

图 2. 光纤随机激光器种子源特性。(a)输出功率与泵浦功率的关系曲线;(b)不同泵浦功率下的光谱;(c) 7.40 W泵浦功率下的短时时域轨迹;(d) 7.40 W泵浦功率下的射频谱
Fig. 2. Characteristics of seed source of RFL. (a) Output power versus pump power; (b) spectra at different pump powers; (c) short-time temporal trace at pump power of 7.40 W; (d) radio frequency spectrum at pump power of 7.40 W
种子源激光输出功率设为0.286 W,然后将其注入至前置放大级中进行放大。前置放大级输出特性如
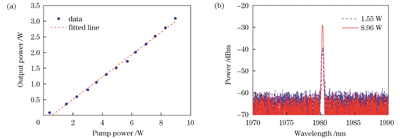
图 3. 光纤随机激光器中前置放大器的输出特性。(a)输出功率与泵浦功率的关系曲线;(b)不同泵浦功率下的输出光谱图
Fig. 3. Output characteristics of preamplifier in RFL. (a) Output power versus pump power; (b) spectra at different pump powers
前置放大级输出的~3.09 W信号光通过MFA注入到主放大级中。主放大级泵浦源为两个高功率的793 nm LD,增益光纤为4.7 m长的LMA-TDF,最终输出百瓦级1980 nm随机激光。主放大级输出功率与注入的泵浦功率的关系曲线如
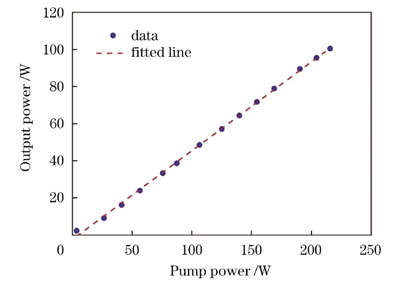
图 4. 光纤随机激光器主放大器中输出功率与泵浦功率的关系
Fig. 4. Output power versus pump power in main amplifier of RFL
光纤随机激光器主放大级的输出光谱特性如
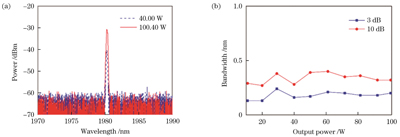
图 5. 光纤随机激光器主放大器的光谱特性。(a)不同输出功率下的光谱;(b)不同输出功率下的3 dB、10 dB带宽
Fig. 5. Spectrum characteristics of main amplifier of RFL. (a) Spectra at different output powers; (b) 3 dB and 10 dB bandwidths at different output powers
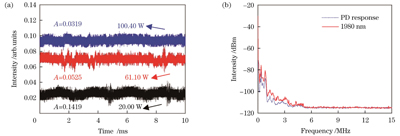
图 6. 光纤随机激光器主放大器的输出特性。(a)不同输出功率下的短时时域强度分布;(b)最大泵浦功率下的射频谱
Fig. 6. Output characteristics of main amplifier of RFL. (a) Short-time temporal intensity distributions at different output powers; (b) radio frequency spectrum at maximum pump power
4 结论
基于MOPA结构的RFL实现了最高输出功率为100.40 W的2 μm光纤随机激光输出。得益于随机激光的独特优势,输出的高功率随机激光具有较窄的线宽:输出功率为100.40 W时3 dB带宽仅为∼0.2 nm,输出激光的短时时域强度波动和长时功率波动均较小,装置具有较高的稳定性。总之,建立的基于MOPA结构的中红外光纤随机激光器实现了高功率窄线宽随机激光输出,为实现2 μm波段高性能激光光源提供了一种解决方案。
[1] Turitsyn S K, Babin S A, El-Taher A E, et al. Random distributed feedback fibre laser[J]. Nature Photonics, 2010, 4(4): 231-235.
[2] Churkin D V, Sugavanam S, Vatnik I D, et al. Recent advances in fundamentals and applications of random fiber lasers[J]. Advances in Optics and Photonics, 2015, 7(3): 516-569.
[3] Gomes A S L, Moura A L, de Araújo C B, et al. Recent advances and applications of random lasers and random fiber lasers[J]. Progress in Quantum Electronics, 2021, 78: 100343.
[4] Wang Z H, Yan P, Huang Y S, et al. An efficient 4-kW level random fiber laser based on a tandem-pumping scheme[J]. IEEE Photonics Technology Letters, 2019, 31(11): 817-820.
[5] Zhang H W, Huang L, Song J X, et al. Quasi-kilowatt random fiber laser[J]. Optics Letters, 2019, 44(11): 2613-2616.
[6] Wang Z N, Wu H, Fan M Q, et al. High power random fiber laser with short cavity length: theoretical and experimental investigations[J]. IEEE Journal of Selected Topics in Quantum Electronics, 2015, 21(1): 10-15.
[7] Vatnik I D, Churkin D V, Podivilov E V, et al. High-efficiency generation in a short random fiber laser[J]. Laser Physics Letters, 2014, 11(7): 075101.
[8] Zhang L, Jiang H W, Yang X Z, et al. Nearly-octave wavelength tuning of a continuous wave fiber laser[J]. Scientific Reports, 2017, 7: 42611.
[9] Wu H, Wang W Z, Li Y, et al. Difference-frequency generation of random fiber lasers for broadly tunable mid-infrared continuous-wave random lasing generation[J]. Journal of Lightwave Technology, 2022, 40(9): 2965-2970.
[10] Leandro D, Rota-Rodrigo S, Ardanaz D, et al. Narrow-linewidth multi-wavelength random distributed feedback laser[J]. Journal of Lightwave Technology, 2015, 33(17): 3591-3596.
[11] Zhang L, Wang C, Li Z Y, et al. High-efficiency Brillouin random fiber laser using all-polarization maintaining ring cavity[J]. Optics Express, 2017, 25(10): 11306-11314.
[12] He J R, Song R, Tao Y, et al. Supercontinuum generation directly from a random fiber laser based on photonic crystal fiber[J]. Optics Express, 2020, 28(19): 27308-27315.
[13] Ma R, Zhang W L, Wang S S, et al. Simultaneous generation of random lasing and supercontinuum in a completely-opened fiber structure[J]. Laser Physics Letters, 2018, 15(8): 085111.
[14] Ma R, Rao Y J, Zhang W L, et al. Backward supercontinuum generation excited by random lasing[J]. IEEE Journal of Selected Topics in Quantum Electronics, 2018, 24(3): 0901105.
[15] Fu Y, Zhu R C, Han B, et al. 175-km repeaterless BOTDA with hybrid high-order random fiber laser amplification[J]. Journal of Lightwave Technology, 2019, 37(18): 4680-4686.
[16] Wu H, Han B, Wang Z N, et al. Temporal ghost imaging with random fiber lasers[J]. Optics Express, 2020, 28(7): 9957-9964.
[17] Ma R, Rao Y J, Zhang W L, et al. Multimode random fiber laser for speckle-free imaging[J]. IEEE Journal of Selected Topics in Quantum Electronics, 2019, 25(1): 0900106.
[18] Wu H, Wang Z N, He Q H, et al. Common-cavity ytterbium/Raman random distributed feedback fiber laser[J]. Laser Physics Letters, 2017, 14(6): 065101.
[19] Ma R, Quan X, Wu H, et al. 20 watt-level single transverse mode narrow linewidth and tunable random fiber laser at 1.5 µm band[J]. Optics Express, 2022, 30(16): 28795-28804.
[20] Quan X, Ma R, Wu H, et al. Low threshold and high spectral purity 1.7 μm random fiber laser based on hybrid gain[J]. Optics & Laser Technology, 2022, 155: 108410.
[21] Mingareev I, Weirauch F, Olowinsky A, et al. Welding of polymers using a 2 μm thulium fiber laser[J]. Optics & Laser Technology, 2012, 44(7): 2095-2099.
[22] Ren X Y, Dai H, Li D T, et al. Mid-infrared electro-optic dual-comb spectroscopy with feedforward frequency stepping[J]. Optics Letters, 2020, 45(3): 776-779.
[23] Hardy L A, Wilson C R, Irby P B, et al. Rapid thulium fiber laser lithotripsy at pulse rates up to 500 Hz using a stone basket[J]. IEEE Journal of Selected Topics in Quantum Electronics, 2014, 20(5): 138-141.
[24] Hao Q, Zhu G S, Yang S, et al. Mid-infrared transmitter and receiver modules for free-space optical communication[J]. Applied Optics, 2017, 56(8): 2260-2264.
[25] Zhang Z, Shen D Y, Boyland A J, et al. High-power Tm-doped fiber distributed-feedback laser at 1943 nm[J]. Optics Letters, 2008, 33(18): 2059-2261.
[26] Yin K, Zhang B, Xue G H, et al. High-power all-fiber wavelength-tunable thulium doped fiber laser at 2 μm[J]. Optics Express, 2014, 22(17): 19947-19952.
[27] Li J F, Sun Z Y, Luo H Y, et al. Wide wavelength selectable all-fiber thulium doped fiber laser between 1925 nm and 2200 nm[J]. Optics Express, 2014, 22(5): 5387-5399.
[28] Yin K, Zhu R Z, Zhang B, et al. 300 W-level, wavelength-widely-tunable, all-fiber integrated thulium-doped fiber laser[J]. Optics Express, 2016, 24(10): 11085-11090.
[29] Zhang Q, Hou Y B, Wang X, et al. 5 W ultra-low-noise 2 µm single-frequency fiber laser for next-generation gravitational wave detectors[J]. Optics Letters, 2020, 45(17): 4911-4914.
[30] Liu Y Z, Cao C, Xing Y B, et al. 406 W narrow-linewidth all-fiber amplifier with Tm-doped fiber fabricated by MCVD[J]. IEEE Photonics Technology Letters, 2019, 31(22): 1779-1782.
[31] Ma R, Liu J, Fang Z Q, et al. Mid-infrared random fiber laser assisted by the passive feedback[J]. Journal of Lightwave Technology, 2021, 39(15): 5089-5095.
[32] Jin X X, Lou Z K, Zhang H W, et al. Random distributed feedback fiber laser at 2.1 μm[J]. Optics Letters, 2016, 41(21): 4923-4926.
[33] Wu H S, Wang P, Song J X, et al. High power tunable mid-infrared optical parametric oscillator enabled by random fiber laser[J]. Optics Express, 2018, 26(5): 6446-6455.
[35] Tian Y, Yao T F, Zhou P, et al. Numerical modeling and optimization of mid-infrared random distributed feedback fiber lasers[J]. Laser Physics, 2018, 28(7): 075104.
[36] Zhou L W, Hu Y Z, Zheng W L, et al. Triple-wavelength thulium-doped fiber random laser based on random fiber grating[J]. Photonics, 2023, 10(4): 355.
[37] 胡耀宗, 朱德才, 黄昌清, 等. 基于随机光栅的掺铥光纤随机激光器研究[J]. 中国激光, 2023, 50(2): 0201002.
[38] Du X Y, Zhang H W, Ma P F, et al. Kilowatt-level fiber amplifier with spectral-broadening-free property, seeded by a random fiber laser[J]. Optics Letters, 2015, 40(22): 5311-5314.
[39] Wang Z H, Yu W L, Tian J D, et al. 5.1 kW tandem-pumped fiber amplifier seeded by random fiber laser with high suppression of stimulated Raman scattering[J]. IEEE Journal of Quantum Electronics, 2021, 57(2): 6800109.
高旺城, 马瑞, 全欣, 陈宇, 范滇元, 刘军. 百瓦级中红外光纤随机激光放大[J]. 中国激光, 2024, 51(5): 0501002. Wangcheng Gao, Rui Ma, Xin Quan, Yu Chen, Dianyuan Fan, Jun Liu. Hundred‑Watt‑Level Mid‑Infrared Random Fiber Laser Amplifier[J]. Chinese Journal of Lasers, 2024, 51(5): 0501002.